Novel Graphene‑Based Nanostructures Production, Functionalization, and Engineering
Manuela Melucc1, Simone Ligi2, Vincenzo Palermo3
1ISOF – Consiglio Nazionale delle Ricerche (CNR), Italy, 2GNext sas, Italy, 3ISOF – Consiglio Nazionale delle Ricerche (CNR), Italy
Introduction
At the beginning of its exciting life, graphene was mostly a game for physicists. Due to the difficulty of producing sufficient amounts of highquality graphene, researchers used single sheets of graphene exfoliated with adhesive tape in very simple geometries to study the outstanding electronic properties of this new material. Ten years after the initial revolution, graphene has gained enormous attention among the general public, being the most “followed” material in online social media and commonly considered as the new wonder material.1
However, major issues hindering the wide application of graphene are still present, including poor processability, and for electronics, the lack of a bandgap in its electronic structure.
More recently, chemists have learned how to “play” with this unique material by enhancing its processability and versatility, and developing different strategies to functionalize and process it. The production of graphene starting from cheap graphite has been demonstrated on a large scale,2 growth of high quality graphene on metallic substrates or on silicon carbide has been optimized, and applications in different fields have been demonstrated at commercial or prototype level.3,4
Our previous works described how the peculiar two-dimensional (2D) shape of graphene gives it unique properties5 such as its interaction with organic molecules in vacuums, liquids, and solid films.6
Using chemistry, it is possible to effectively tailor this poorly soluble and almost chemically inert material into a wide variety of forms ranging from simple, soluble sheets to hierarchical architectures where 2D graphene sheets are assembled into three-dimensional (3D) bulk materials or foams for applications in electronics, composites, energy storage, or catalysis.
Graphene Chemistry in Solution at Single-sheet Level
The simplest way to process and tailor graphene is to work with single sheets in solution. Production on a large scale can be achieved either using “soft” approaches, i.e., non-covalent supramolecular interactions that do not damage the graphene lattice,7–9 or “harsh” chemical approaches, i.e., oxidation to graphene oxide (GO) and subsequent reduction that inevitably results in chemical defects on the surface of the sheets (Figure 1).10–12
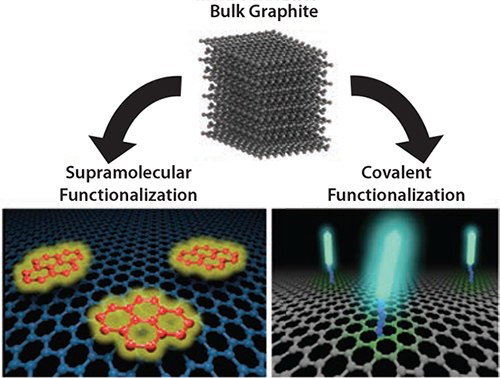
Figure 1.Schematic of different approaches to exfoliate graphite and functionalize graphene by using covalent or non-covalent (supramolecular) approaches.
GOs (Prod. Nos. 763705, 777676, 794341, etc.) have long been the underdog of the graphene family due to their chemical defects that diminish many of the unique physical properties of graphene. However, these same characteristics make GOs highly processable, versatile, and water soluble. Thermal, chemical, or electrochemical reduction can be used to re-establish conductivity, but some damage to the lattice always remains, giving reduced graphene oxide (RGO) (Prod. No. 777684) much less performance than graphene. Nevertheless, production and successful application of different forms of GO and RGO have been demonstrated;13–20 some of them seem even closer to commercial applications than the most advertised applications of graphene for electronics or transparent conductors.
The exfoliation of graphite (Prod. Nos. 496588, 496596, etc.) to produce graphene is a complex phenomenon that takes place on a nano- and mesoscopic scale. Similar to other solubilization processes, the process depends on a complex competition between chemical, electrostatic, and Van der Waals interactions,21 as well as on fluid dynamics at the micro-scale. Recently, we conducted a systematic and comparative study on exfoliated graphene produced using mechanical, chemical, and electrochemical approaches.22 Among the three methods, chemical oxidation to produce GO was found to be a very effective but disruptive method to exfoliate graphite. Electrochemical oxidation, which uses electric fields to drive molecular intercalation in graphite, allows for a fast exfoliation and in-depth disruption of bulk quantities of graphite, but also results in damage to the resulting graphene lattice.
The supramolecular exfoliation approach, based on sonication in organic solvents, yields graphene of the best quality, but due to the high-energy input requirements of the sonication process, sheets with low lateral size (typically <1 μm) are obtained. The differences observed from the three methods demonstrate the existing trade-off between speed and efficiency of exfoliation on one side, and preservation of material quality on the other.22
Once exfoliated in solution, graphene sheets can be chemically modified (both covalently and non-covalently) using a variety of molecules or nano-objects to yield new, property-specific, tailored, graphene-based materials.23 The highly conjugated sp2 carbon structure of graphene gives it a strong affinity to polyaromatic organic materials, such as organic semiconductors or industrial dyes characterized by extended π-electrons delocalization. These molecules, unlike graphene, have excellent processability and tunable HOMO, LUMO, and bandgap.6
This affinity is currently being investigated in order to create grapheneorganic hybrids with enhanced solubility in water or organic solvents, good dispersibility in polymer composites and/or novel optoelectronic functions. Although it is difficult to give a fair and exhaustive overview on all the graphene-based hybrid systems developed so far, interesting examples include hybrids based on organic materials, as well as metal nanoparticles and biomolecules, even DNA. Most common moieties used are perylenes (Prod. No. 394475, etc.),9,24,25 pyrenes (Prod. No. 571245, etc.),8,26-29 porphyrins (Prod. No. 252921, etc.),30–34 fullerenes (Prod. No. 572500, etc.),35 oligothiophenes (Prod. No. 691631, etc.),36,37 polythiophenes (Prod. No. 698997, etc.),38,39 metal nanoparticles,40,41 or even biological moieties.42,43
There are several empirical models that use the surface tension of the solvent or solubility parameters to predict the dispersion of graphene in different solvents.7 However, there is still no clear understanding at the molecular level why some molecules interact with graphene more effectively than others. We conducted a systematic study to compare the efficiency of several pyrenesulfonic acid sodium salts (Prod. Nos. 82657, 37920 and 82658, and Prod. No. H1529), the watersoluble pyrene moieties, as exfoliaton agents for the preparation of stable aqueous suspensions using liquid exfoliation.8 These pH-sensitive derivatives are not only interesting for fundamental research, but also for practical applications due to their high photoluminescence quantum yield, excellent water solubility, and lack of toxicity. They also have largescale, commercial applications in highlighters, pencils, and soaps.
We examined a series of four pyrene derivatives featuring an increasing number of polar groups and found all of them exfoliate graphite, yielding stable suspensions of graphene in water. A relevant fraction of monolayer graphene sheets covered by a layer of pyrene molecules was obtained.26 The total concentration of suspended graphene depends significantly on the number of polar groups on the hydrophobic pyrene core. Molecular dynamic calculations revealed that a critical factor in the interaction of pyrene derivatives with graphene involves a thin layer of solvent molecules, confined between the pyrene molecules and the graphene surface. The amphiphilic pyrenesulfonic molecule changes its orientation when approaching the surface to slide into this layer in a most effective way for molecules with an asymmetric shape showing a strong dipole. Simulations indicate the molecular dipole is not important per se, but it facilitates the “sliding” of the molecule into the solvent layer, resulting in the lateral displacement of the water molecules located between the aromatic core of the pyrene dye and the graphene substrate.
The interaction of organic molecules on graphene sheets at the nanoscale is not only of great interest from the fundamental point of view to better understand and improve graphene exfoliation,8 but also has important implications for advanced applications. Thus, the pyrene-based method to exfoliate graphite with a well-established industrial dye was extended to process graphene in polymers.45 The same pyrenesulfonic acids have shown a similar ability to solubilize other 2D materials such as boron nitride (Prod. Nos. 255475 and 790532), tungsten disulfide (Prod. Nos. 243639 and 790583), molybdenum sulfide (Prod. Nos. 234842 and 69860), selenides, and tellurides (Figure 2). The materials can be used to fabricate a photodetector device based on different layers of 2D materials.44

Figure 2.A) Chemical formula of four pyrene derivatives compared for supramolecular graphite exfoliation. B) Optical images of the as-prepared 2D crystal-based dispersions using pyrene derivatives (the numbers 1, 2, 3 and 4 refer to the number of sulphonic groups of the organic dyes shown in (A).8,44
In parallel to non-covalent modification approaches based on supramolecular interactions, different synthetic methods have been proposed worldwide to covalently bind functional organic materials onto graphene and graphene oxide. The development of more efficient and controlled synthetic protocols to achieve this aim is a challenging issue. Given the poor dispersibility and intrinsic inert chemical structure of graphene, its reactivity is much more limited than that of GO, and most mainly rely upon the formation of covalent bonds between free radicals or dienophiles and C=C bonds.46,47 Conversely, GO can undergo the reactions typical of carboxylic, carbonyl, epoxide, and hydroxyl functionalities; thus, it is easier to modify than graphene. Among the most common synthetic methods for GO functionalization is the activation of the carboxylic moieties followed by reaction with nucleophilic groups (i.e., amines37). One recent proposed alternative for GO functionalization relys on silylation of surface oxydrilic groups promoted by MW irradiation. This method can be used to graft trialkoxysilane ended π-conjugated chromophores on GO sheets surface.36
Several authors have studied how the properties of functional molecular materials influence the behavior of graphene after physical doping48–50 or covalent engrafting.37,51 At the same time, the question of how the properties of the molecule will be affected by the presence of graphene remains. It is well-known that graphene and GO strongly quench the photoemission of light-emitting molecules,52–54 but how much is this interaction influenced by the nanoscale structure of this graphene-organic ensemble? By engineering the structure of the system at the nanoscale, it is possible to tune this interaction. For example, low quenching (~16%) is observed when oligothiophene dyes are tethered to GO using flexible alkyl linkers,37 stronger quenching (~60%) is achieved with shorter linkers,51 and nearly complete quenching is attained by depositing GO on a self-assembled monolayer of the same oligothiophenes (Product Nos. 547905 and 594687)52 (see Figure 3A).
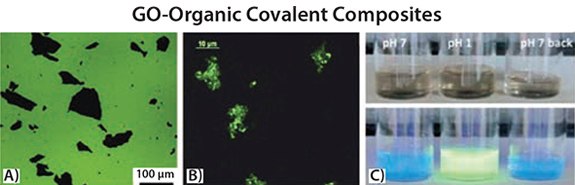
Figure 3.A) GO sheets quenching the fluorescence of a thin layer of oligothiophene molecules on SiOx.52 B) Fluorescent GO sheets, obtained by functionalization of GO with oligothiophene dyes, using a flexible diamine linker.37 C) Images of GO–oligothiophene covalent composites in EtOH at neutral pH, upon acidification with HCl and re-neutralization by triethylamine (TEA) (from left to right) under normal light (top) and UV irradiation (bottom) showing the reversible emission switch.
Besides fluorescence quenching, the same oligothiophene–GO ensemble was used to study how tethering the molecule to GO affects its chemophysical properties. Molecules will behave differently when isolated in a solvent ather than tethered to a bulk, macroscopic surface. However, molecules tethered to graphene or GO are in an ambiguous situation since the size of a 2D sheet is much larger than that of a typical molecule. This is, in fact, more comparable to attachment to a bulk conventional surface but simultaneously dissolved in solution.5 We examined the interaction of GO with a terthiophene dye using pH-sensitive fluorescence emission to determine how the emission changes when the molecules are free in solution or tethered to a GO (Figures 3B–C). We found that covalent attachment to GO does not perturb the absorption and emission properties of the dye and, in particular, the pH sensitivity.
From Solution to Real Materials
Once the 2D sheets of graphene (or chemically modified graphene) have been processed and functionalized in solution (either by exploiting supramolecular interactions or by passing through graphene oxide), it is very easy to deposit them on silicon substrates with micro/nano electrodes to create devices such as transistors or sensors. Taking advantage of the high processability and easy charge transfer between graphene and organic materials, it is possible to prepare devices with tunable percolation that allow it to merge the good charge mobility of RGO with the semiconducting behavior of organic materials.
Some recently published examples include RGO/poly(3-hexylthiophene) bilayers,18 graphene/phenyloctane, and graphene/arachidic acids blends;55 and graphene blends with high-performing semiconductive polymers, like poly[N,N-9-bis(2-octyldodecyl)-naphthalene-1,4,5,8-bis(dicarboximide)-2,6-diyl]-alt-5,59-(2,29-bithiophene)], and (P(NDI2OD-T2)).56
The graphene and the organics can be deposited simultaneously to form a thick intermixed blend,55,56 or sequentially to form a bilayer.18
Utilizing the ability of organic π-conjugated molecules to exfoliate graphite,8,55 graphene exfoliation can be performed in the same solution used for the organic material deposition on devices. Alternatively, an ex situ two-stage approach based on more “classical” solvents to exfoliate graphite and subsequent mixing with the organic semiconductor can be used.56 By using the suitable solvents and applying electric fields, it is possible to deposit the sheets selectively on the source, the drain, or in between the electrodes.57,58
Processing Graphene in More Complex, Multilayer Structures
In addition to simple mixtures or bilayers with semiconducting polymers, more complex structures can be constructed by taking advantage of the enhanced processability and tunability of chemically modified graphene.
Multilayer “sandwich” structures can be engineered using high-quality graphene alternated to self-assembled monolayers of 1-pyrenebutyric acid N-hydroxysuccinimide using layer-by-layer (LbL) deposition. Such multilayered structures showed an enhancement of six orders of magnitude in electric conductivity, as compared to pure graphene, due to graphene doping by pyrene.59
In a similar approach, multilayer structures of GO alternated to poly-llysine can be created using LbL. Heating to 800 °C, the GO is reduced and doped with poly-lysine, yielding micro-supercapacitors with an ultrahigh volumetric capacitance of ~488 F/cm3 and an outstanding rate capability of up to 2,000 V/s.
GO-based structures with 2D porosity can be obtained using covalent functionalization with linkers of variable length. For example, the p-xylylenediamine intercalation reaction of graphene oxide (GO) is a convenient and simple approach to prepare pillared-type graphenebased materials.60 The generation of such a constrained system (covalently ‘‘stitched’’) along the graphene c-axis allows it to considerably change the interlayer distance when compared to pristine GO, thanks to a crosslinking process of the p-xylylenediamine (Prod. No. 279633) with the epoxide surface groups of adjacent GO sheets. The increased GO interlayer distance provides materials with increased porosity and specific surface areas up to five times higher than those of pristine GO.
A more coarse-grained structure has been obtained creating multilayers of solvent-exfoliated graphene sheets and thin layers of vacuum-sublimed metallic tin. Upon annealing at 300 °C in Ar/H2, the tin layer breaks down into isolated nanopillars that act as spacers for graphene and as an ion reservoir for lithium storage in batteries (Figure 4A).61
The as-formed 3D multilayered nanostructure was directly used as an anode material for rechargeable lithium-ion batteries without adding any polymer binder or carbon black. Electrochemical measurements showed very high reversible capacity and excellent cycling performance at a current density as high as 5 A g–1. This is a good example of how novel structures can be obtained by combining liquid processing with vacuumbased techniques such as thermal evaporation.
In another example, highly uniform sandwiches of GO sheets embedded in porous carbon (PC) were obtained using ionic liquid functionalized graphene-oxide sheets as a shape-directing agent and a resorcinol/formaldehyde polymer as the carbon precursor. The PC/GO/PC multilayer sandwich structures obtained show excellent rate capability, a high specific capacitance of 341 F g–1 at 5 mV s–1, and good cycle stability over 35,000 cycles without any conductive additive.
The production of graphene composites at single-sheet level in solution or in very thin layers on flat substrates, has been described mostly for electronics applications. Moving to an even larger scale, more complex structures can be constructed by using mesoscopic templates to obtain bulk, macroscopic hierarchical materials.
Increasing Complexity Up To the Macroscale: Foams for Energy Storage or Catalysis
By experimenting with the oxidation, reduction, and/or chemical functionalization of GO, 2D sheets of different kinds can be processed or even directly grown on virtually any micro- or nano-porous templates like metallic62,63 or polymeric foams,64 inorganic ZnO nanocrystals,65 or waterorganic liquid interfaces in emulsions.66,67 Graphene can be used to wrap or coat other nanostructures68 or even electrodes.58 Conversely, its surface can be coated with other materials acting as a 2D substrate to support less conductive or less robust materials.69,71
These architectures are often visually beautiful (Figures 4–5), but also interesting from the technological point of view because they can be further functionalized with other materials having properties synergistic and complementary to those of graphene.
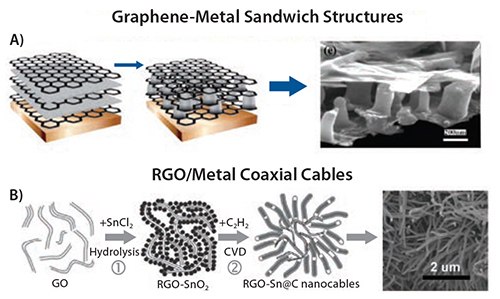
Figure 4.A) Schematic of the graphene/Sn-nanopillar nanostructure preparation procedures, and corresponding SEM image.61 B) Schematic of procedures for the synthesis of RGO-supported Sn/C nanocables, and corresponding SEM image.
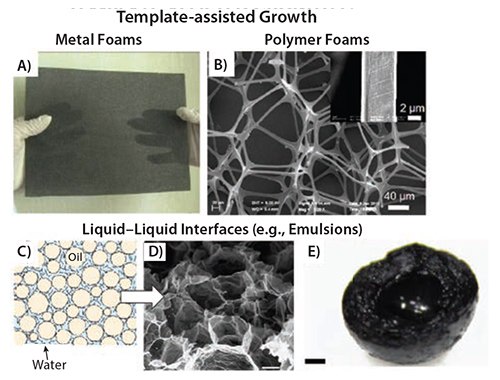
Figure 5.Graphene-based 3D structures templated using A) metal foams,62 B) polymeric foams,64 or C–E) in liquid emulsions.66
Graphene has outstanding electrical, optical, mechanical, and thermal properties, but contrary to the media hype, it can’t be good at everything. Some other materials have better properties for energy applications; Fe2O3 is a promising low-cost, abundant, and non-toxic candidate for pseudocapacitor electrodes with high theoretical capacity (1,007 mA h g–1), much better than that of commonly used graphite-based materials (372 mA h g–1). However, the practical applications of Fe2O3-based electrodes are limited due to their low electrical conductivity and inferior cycling stability. Thus, the combination of graphene and Fe2O3 is of high interest for the development of next-generation batteries.
We produced hierarchical graphene-based composites with Fe2O3 for energy storage using a combination of electrochemical and solutionprocessing techniques. Electrochemically exfoliated graphene oxide (EGO) sheets were produced using a custom-built setup that allowed fast expansion of graphite flakes and efficient exfoliation of expanded graphite via an electrochemical route. The resulting sheets were deposited on sacrificial nickel foam together with an iron hydroxide colloidal precursor. Subsequent calcination treatment simultaneously rendered the EGO foam conductive and transformed Fe(OH)3 into hematite (α-Fe2O3), yielding a nanoporous Fe2O3 layer on the surface of the mesoporous EGO foam. The resulting graphene/metal oxide hybrid was a continuous, electrically conductive 3D composite, that made an ideal structure for lithium storage (Figure 6), featuring a hierarchical meso-nano porous structure (Figures 7A–D). By using liquid processing, the nanostructured material can be optimized by tuning the Fe2O3:EGO ratio in order to maximize performance as standard coin cell battery electrodes.63
With the decoration of Fe2O3 nanoporous coatings, the initial discharge capacities can be enhanced up to 701 mAh g–1, a value comparable to commercially available batteries, and a higher energy capacity can be retained after the first discharge/charge cycle (Figure 7E).
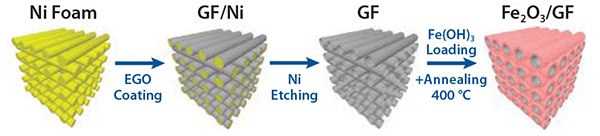
Figure 6. Schematic of template-assisted deposition of electrochemically exfoliated graphene oxide (EGO) on a nickel metallic foam, and successive reduction to give conductive foams (GF), either uncoated or coated with iron oxide (hematite).
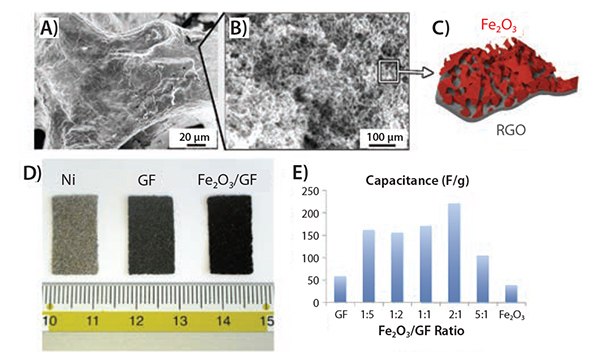
Figure 7. A) SEM image of a mesoporous, conductive graphene foam coated with a nanoporous layer of iron oxide. B) Inset of A). C) Illustration showing the hierarchical structure of the Fe2O3/GF porous layer. D) Photograph comparing the original Ni foam, the GF and the Fe2O3/GF samples. E) Specific capacitance values obtained varying Fe2O3:GF ratio.63
In addition to energy storage, catalysis is another promising field where graphene-based porous structures can be used. In particular, graphenebased materials have shown promise as efficient cathode catalysts for the oxygen reduction reaction (ORR), a critical process in fuel cells.
Fe3O4/graphene foams show a more positive onset potential, higher cathodic density, and higher electron transfer numbers for the ORR in alkaline media than Fe3O4 nanoparticles supported on more conventional materials (N-doped carbon black or N-doped graphene sheets). This improvement can be attributed to the role of the 3D macropores and the high specific surface area of the graphene-based support for improving the ORR performance.69 A more detailed review on the use of porous graphene materials for advanced electrochemical energy storage and conversion can be found in Reference 72.
Conclusions
The field of graphene chemical exfoliation, processing, and functionalization is currently bursting with several scientific results being published every month. It is expected this trend will accelerate even more in the next years as graphene with standardized quality, well-controlled properties, and low cost becomes more widely available for industrial applications all over the world. Affordable prices are already claimed for powders of multi-layer graphene and applications that use graphene based composites are already available to the end-user market. Moreover, chemical functionalization can enhance the interaction of graphene with polymeric matrices in composites to improve mechanical properties while allowing for the use of very low amounts of graphene.
Even more than its outstanding electronic properties, the chemical versatility and processability of graphene enables production of an exceptionally wide range of materials with advanced and unprecedented functionalities. In fact, the field of chemistry itself is experiencing a graphene-stimulated revival. Conventional organic chemistry methods need to be properly readapted for graphene tailoring, representing a challenging issue for organic chemists. In parallel, novel ad hoc protocols and reactions have to be developed for the realization of different composite materials: from simple graphene sheets coated by a thin layer of molecules, to bulk composites of graphene and organic materials, to more complex forms where graphene acts as a 3D, robust, and versatile scaffold to be combined with organic, inorganic, or even biological materials.
Acknowledgments
The research leading to these results has received funding from the European Union Seventh Framework Programme under grant agreement n°604391 Graphene Flagship, the EC Marie-Curie ITN- iSwitch (GA no. 642196). The project UPGRADE acknowledges the financial support of the Future and Emerging Technologies (FET) programme within the Seventh Framework Programme for Research of the European Commission, under FET-Open grant number: 309056. We acknowledge the Operative Program FESR 2007-2013 of Regione Emilia-Romagna – Attività I.1.1.
References
如要继续阅读,请登录或创建帐户。
暂无帐户?