Dihydropinene (DHP, Pinane): A Bio-based Versatile Greener Solvent for Organic Synthesis
Saikat Sinha1, R&D Lead, customer solution R&D
Markus Obkircher1, Head of customer solutions R&D
Rhushikesh Deokar1, Senior Scientist I R&D
Senthil Kumar MD1, Scientist II R&D
Fernando Luengo2, Lead Expert, Product & Portfolio Management
1Sigma-Aldrich Chemical Pvt. Ltd. (Bangalore, India)
2Millipore Sigma (Milwaukee, United States)
Abstract
It is well accepted that solvent losses and emissions from chemical reactions/processes lead to environmental pollution, hence the development of more sustainable, non-volatile and recyclable greener solvents for different applications is of great importance. This application note overviews some recent studies in organic synthesis using the greener alternative solvent dihydropinene.
Section Overview:
Introduction
The use of common organic solvents derived from non-renewable energy sources1 (oil, gas and other fossil fuels) are facing increasing restrictions in terms of chemical safety and environmental regulations. Thus, seeking greener alternatives to replace them is of urgent need. The fully bio-based and biodegradable2 solvent dihydropinene (DHP, pinane) has emerged recently as an alternative option.3
Dihydropinene (DHP, pinane) is a set of isomeric terpenes (shown in Figure 1). Both the cis and trans forms of this solvent demonstrate usefulness in several chemical transformations as a greener alternative solvent compared to other traditional solvents such as toluene, hexane, dichloromethane, and benzene. DHP has structural similarities to cyclohexane in having a six-membered aliphatic core, however, it also contains an additional alkane bridge. Dihydropinene is derived from pinene, the major component of the abundant and cheap turpentine pine tree oil produced worldwide in large quantities by catalytic reduction.4 Due to its structural similarity to cyclohexane and more sustainable chemical feedstock, dihydropinene has been considered as a substitute for fossil-based hexane in the academic literature.5
DHP has a higher viscosity6a-c and boiling point than hexane (Table 1), which may limit its use as a replacement for traditional solvents sourced from fossil fuels, especially in regard to chemical synthesis. However, these same properties-higher boiling point and viscosity offer distinct advantages for recycling. The higher boiling point contributes to low volatility, reducing solvent loss during recycling processes, and facilitates separation from reaction products and solvents with lower boiling points. This makes it potentially well-suited for use in scale-up and process development, especially when one also considers its sustainability credentials. Additionally, like toluene, dihydropinene forms an azeotrope with water, providing benefits for certain synthesis strategies. Beyond its role in synthesis, DHP is frequently employed as a solvent for oil extractions.7

Figure 1.Isomers of dihydropinene (DHP, pinane)
CAS Number: | 473-55-2 (mixture of isomers) |
---|---|
Molar Mass: | 138.25 g/mol |
Chemical Formula: | C10H18 |
Boiling Point: | 169 °C |
Flash Point: | 40 °C |
Density at 20 oC: | 0.85-0.87 g/mL |
Viscosity: | 1.3 mPa·s (cP) at 298.15 K |
Refractive Index (nD): | 1.4633 at 298.15 K |
Experimental
This study presents several reaction examples to assess the potential of dihydropinene (DHP) as a greener solvent, emphasizing its versatility in a range of chemical transformations and its capacity to replace non-renewable, more toxic solvents. A comparison overview of procedures and yields is shown in Table 2. The reactions selected for evaluation span a wide array of chemical transformations, demonstrating DHP’s flexibility and its potential to replace conventional solvents, such as benzene and toluene, which are often harmful.
The present study includes reactions such as halogenation and N-alkylation, which are common methods for introducing functional groups and performing nucleophilic substitutions. DHP’s ability to serve as a greener alternative to these toxic solvents highlights its applicability across these transformations. Additionally, the Suzuki coupling reaction is used to evaluate DHP as a solvent in a key palladium-catalyzed process, indicating that DHP can effectively replace toluene in cross-coupling reactions, which are essential for both advanced material synthesis and are often used in the synthesis of complex drug molecules. Advanced synthetic strategies, such as Diels-Alder cycloaddition and ring-closing metathesis, further demonstrate DHP’s compatibility with pericyclic and catalytic reactions. Through these diverse examples, ranging from carbon-carbon bond formation to catalytic and nucleophilic processes, DHP proves to be a more sustainable alternative to traditional solvents. The experimental findings of this comprehensive evaluation, summarized in Table 2, highlight DHP’s efficiency and suitability for a broad range of chemical syntheses. For more detailed conditions used for the shown reactions, refer to the appendix.
Condensation Reactions
Condensation reactions are pivotal in synthetic chemistry and material science, driving advancements in drug discovery, polymer chemistry, natural product synthesis, and materials science. They enable the creation of complex molecules, polymers, and functional materials, providing essential tools for innovation in research and industrial applications.
Knoevenagel Condensation
The Knoevenagel condensation is a modified Aldol condensation that involves the formation of an α, β-unsaturated carbonyl compound through the condensation of an aldehyde or ketone with a compound containing an activated methylene group in the presence of base. The reaction is commonly used to construct building blocks used in the dyes and polymers, therapeutic drugs, flavors, and perfumes fields, among others8. This reaction often uses hazardous solvents such as DMAc, DCM, DMF, toluene, and/or pyridine.8 There are few reports using greener approaches [such as ionic liquid9, water10 or solvent-free11], however, these are yet to have found wide adoption. Dihydropinene is an alternate more sustainable reaction solvent, with some useful properties warranting further investigation of its use on the industrial scale. In the below example (Figure 2) 4’-chloro acetophenone (C19708) was condensed with malononitrile (M1407), in the presence of ammonium acetate (238074) and acetic acid (695092) in DHP as a solvent under heating conditions to yield 2-(4-chlorophenyl)malononitrile (1).

Figure 2.Synthesis of 2-(4-chlorophenyl)malononitrile (1) through Knoevenagel condensation12 of 4’-chloro acetophenone (C19708) and malononitrile (M1407) in pinane.
Thalidomide Synthesis
Thalidomide and related compounds are a very interesting class of compounds13 commonly found in many therapeutic drugs. It became a valuable core for compounds used for treating multiple myeloma and leprosy-related conditions due to its immunomodulatory and anti-inflammatory properties, and it plays a key role in targeted therapies like PROTAC technology.14a,b The structure, featuring a phthalimide core and glutarimide moiety, highlights the importance of stereochemistry and functional groups in drug activity, such as pomalidomide, a chemotherapy drug. Synthesis of the core structure thalidomide (T144) in dihydropinene (DHP) afforded a comparable yield from the condensation of phthalic anhydride (320064) and 3-aminopiperidine-2,6-dione hydrochloride (2) in DHP-AcOH solvent (Figure 3).
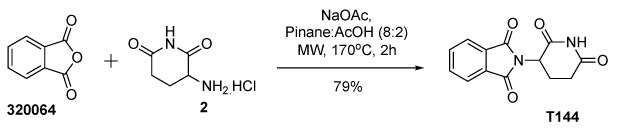
Figure 3.Synthesis of thalidomide (T144) from condensation of phthalic anhydride (320064) and 3-aminopiperidine-2,6-dione in DHP-AcOH mixture.
Substitution Reactions
Substitution reactions are essential in the discovery of new molecules, particularly in medicinal chemistry, pharmacology, materials science, and synthetic chemistry. These reactions facilitate the modification of chemical structures, optimizing properties such as biological activity, solubility, selectivity, and stability. This application note highlights the versatility of dihydropinene in a number of substitution reactions, including halogenation and O-/N-alkylation, which are commonly employed in laboratories. In our studies, we explored the use of dihydropinene (DHP) as a more sustainable alternative to traditional non-renewable solvents such as benzene,15a toluene,15b and methanol15c. The findings underscore DHP's capability to replace conventional solvents while maintaining efficient yields, supporting its adoption in various industrial and laboratory applications.
Halogenation using Dihydropinene
Tetraethyleneglycol (110175) was converted to bis[2-(2-chloroethoxy)ethyl]ether (14625) with thionyl chloride (230464) by heating with DHP in the presence of pyridine (270970) as depicted in (Figure 4).
![Halogenation using Dihydropinene The chemical synthesis pathway for Bis[2-(2-chloroethoxy)ethyl]ether, starting from tetraethyleneglycol and thionyl chloride in dihydropinene. The process begins with the molecular structure of tetraethyleneglycol, depicted with two terminal hydroxyl groups. The reaction involves thionyl chloride, indicated by its chemical formula, and is carried out in the presence of pyridine and dihydropinene as solvents. The conditions specify room temperature and a reaction time of 20 hours, resulting in a 73% yield. The final product, Bis[2-(2-chloroethoxy)ethyl]ether, is shown with two terminal chloride groups replacing the original hydroxyl groups, completing the transformation.](/deepweb/assets/sigmaaldrich/marketing/global/images/technical-documents/articles/chemistry-and-synthesis/reaction-design-and-optimization/figure-4-halogenation-using-dihydropinene/figure-4-halogenation-using-dihydropinene.jpg)
Figure 4.Synthesis of Bis[2-(2-chloroethoxy)ethyl]ether (14625) from tetraethyleneglycol (110175) and thionyl chloride (230464) in dihydropinene.
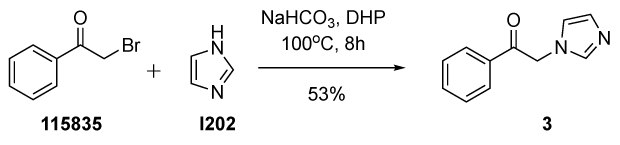
Figure 5.Synthesis of 2-(1H-imidazol-1-yl)-1-phenylethanone 3 from 2-bromoacetophenone (115835) and imidazole (I202) in dihydropinene.
Coupling Reactions
Cross-coupling reactions are valuable synthetic tools used for the preparation of a wide variety of organic compounds, from natural and synthetic bioactive compounds to new organic materials, in all fields of chemistry.16 Regulatory restrictions affecting some the most frequently used traditional solvents for cross-coupling reactions are driving interest in greener alternative solvents.17 NMP, DMF, 1,4-Dioxane, toluene, MeCN, THF, and dimethoxyethane are among the most popular solvents for cross-coupling reactions. There have been investigations of more sustainable solvents in cross-coupling reactions, such as the more sustainable solvent selection guide, which was utilized in the Suzuki–Miyaura coupling of amides by Peng Lei et. al.18 replacing conventional solvents with greener solvents like tert-butyl ether (MTBE), cyclopentyl methyl ether (CPME), diethyl carbonate (DEC), p-cymene, dimethylcarbonate (DMC), and anisole. In a similar line, we demonstrated our efforts to use dihydropinene as a greener substitute for the conventional solvents.
Suzuki Palladium-Catalyzed Cross Coupling Reaction
Herein, an alternative greener solvent composition consisting of DHP-ethanol-water has been demonstrated to replace conventionally reported solvents.19 4-(furan-2-yl)benzaldehyde (4) was synthesized from the coupling of 4-bromo benzaldehyde (B57400) and 2-furan boronic acid (464910) in presence of the precatalyst tetrakis(triphenylphosphine)palladium(0) (216666) and DHP:ethanol:water mixture (50 mL, 2:2:1) as a solvent mixture (Figure 6). Here, DHP has been used to substitute the petroleum-based solvent toluene.
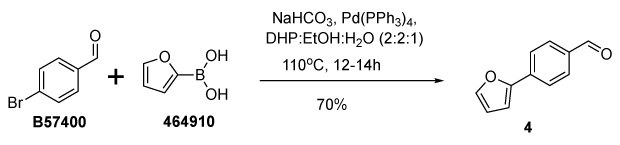
Figure 6.Synthesis of 4-(furan-2-yl)benzaldehyde (4) from 4-bromo benzaldehyde (B57400) and 2-furan boronic acid (464910) in pinane:ethanol:water mixture.
Cyclization Reactions
Cyclization reactions are critically important in discovery processes across a wide range of scientific fields, including drug discovery, material science, natural product synthesis, and photochemistry. Their ability to efficiently create complex cyclic structures with high selectivity and stereochemistry makes them indispensable in the development of bioactive compounds, advanced materials, and functionalized polymers. By enabling the creation of novel scaffolds, bioactive molecules, and innovative materials, cyclization reactions continue to play a central role in advancing both fundamental science and applied technology.
Diels-Alder Reaction
A reaction between a conjugated diene and a substituted alkene (dienophile) produces a cyclic product in the Diels-Alder (DA) reaction. Over the last few decades, DA and its complementary retro variant have found wide use in industry for synthesizing pharma grade products such as vitamin D and its analogues, abacavir, isopyrazam, ifetroban sodium, biperiden, provitamin K3, vitamin B6.20 It is also commonly used in total synthesis, with streptonigrone, ipalbidine, (+)-reserpine, prostaglandins, phyllantine, etc. being notable natural products synthesized by various research groups using the Diels-Alder reaction as a key step21. As these reactions are already atom economical, we aimed to provide more sustainability value by replacing the traditional solvents with DHP.
Anthracene (141062) is reported to undergo Diels-Alder cycloaddition reaction with maleic anhydride (M188) to afford the cycloaddition adduct dibenzo(h,k)-4-oxatricyclo(5.2.2.02,6)undec-8,10-diene-3,5-dione (S958166) in the solvents toluene and xylene, which are derived from the non-renewable resource petroleum.22a,b Here the more sustainable DHP has been used to substitute the fuel-sourced solvent toluene for the reaction depicted in Figure 7. When this reaction is carried out using DHP, we are able to recover ~90% of DHP by distillation from the batch and recycle it for another batch of the reaction.
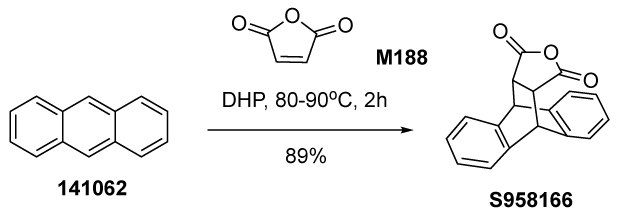
Figure 7.Diels-Alder (DA) cycloaddition of anthracene (141062) and maleic anhydride (M188) in dihydropinene.
Ring Closing Metathesis
Olefin metathesis reactions catalyzed by ruthenium complexes continue to be of great importance for the synthesis of various unsaturated rings and heterocycles via the intramolecular ring-closing metathesis (RCM) of two terminal alkenes. The reaction has been widely used in a variety of applications, including polymer chemistry, drug discovery, and chemical biology.23 Experimental studies by several groups have identified solvent influences on both rates as well as turnover frequencies of metathesis reactions.24 Solvents commonly used for RCM reactions, are mostly derived either from fossil fuels or hazardous. With a few exceptions, the majority of metathesis reactions are carried out in halogenated and aromatic solvents, which are toxic and harmful (dichloromethane and 1,2-dichloro-ethane belong to ICH class 1 and toluene to ICH class 2 solvents)25. The use of some of these solvents is already limited or restricted. In the below example, diethyl diallylmalonate (283479) is reported for facile ring-closing metathesis yielding the corresponding cyclopentene (5).26 We’ve found DHP as a suitable alternative to DCM, benzene, and toluene, which are very commonly used as solvents for ring-closing metathesis and specifically DHP is replacing DCM in this particular conversion (Figure 8) with a comparable yield (Table 2).
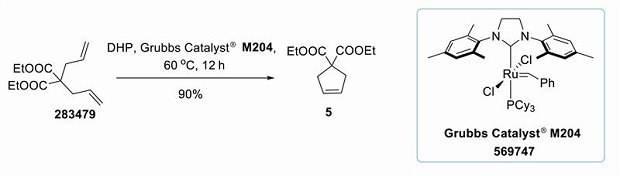
Figure 8.Grubbs catalyst® M204 (569747) mediated ring closing metathesis of diethyl diallylmalonate (283479) in dihydropinene.
Discussion
The experimental study demonstrates the effectiveness of dihydropinene (DHP) as a more sustainable, bio-based solvent in a variety of chemical reactions as summarized in (Table 2). These include condensation, substitution, cross-coupling, cyclization, and ring-closing metathesis. DHP consistently achieved comparable or superior yields to conventional fossil-fuel-derived solvents such as toluene, DMF, and dichloromethane.
Reaction Type | Reported in Literature | Experimental findings using DHP | |||||
---|---|---|---|---|---|---|---|
Solvent | Time | Temperature | Yield (%) | Time | Temperature | Yield (%) | |
Knoevenagel condensation | Toluene11 | 24 h | 105 °C | 95 | 4 h | 120 °C* | 82 |
Thalidomide synthesis | DMF14a,b | 18 h | 120 °C | 69 | 2 h | 175 °C* | 79** |
Halogenation | Benzene15a | 27 h | Ambient | 76 | 20 h | Ambient | 73 |
N-Alkylation | Toluene15b | 20 h | 115 °C | 58 | 8 h | 100 °C | 53 |
MeOH15c | 2 h | 65 °C | 70 | ||||
Suzuki coupling | Toluene19 | 15 h | 115 °C | 47 | 14 h | 110 °C | 70 |
Diels Alder reaction | Xylene22a | 45 min | 150 °C | 89 | 2 h | 80-90 °C | 89** |
Toluene22b | 48 h | 90 °C | 90 | ||||
Ring closing metathesis | DCM25 | 1 h | Ambient | 91 | 12 h | 60 °C** | 90 |
* Temperature has been elevated for reaction kinetics and effective mixing for more viscous dihydropinene. ** Reaction with recycling of DHP after completion of the reaction. |
DHP has demonstrated its efficiency as a solvent in performing these specific examples of key transformations like Knoevenagel condensation, N-alkylation and halogenation with comparable yields of 82%, 53%, and 73% respectively. Moreover, in the thalidomide synthesis and the Suzuki cross-coupling reaction, DHP showed improved yields of 79% and 70% respectively, surpassing yields from traditional solvents. When used in the Diels-Alder cycloaddition and ring-closing metathesis reactions, DHP achieved equivalent yields to those of traditional solvents. Apart from being a greener substitute for traditional solvents, DHP also offers benefits in recyclability and environmental compatibility.
Conclusion
The report discusses dihydropinene (DHP) as a bio-based, biodegradable solvent that can serve as a greener alternative to traditional organic solvents derived from fossil fuels. The above finding highlights DHP's effectiveness in various chemical transformations, including Knoevenagel condensation, thalidomide synthesis, and Suzuki coupling, achieving comparable yields to conventional solvents. The report underlines the suitability of DHP for more sustainable chemistry practices, emphasizing its potential to reduce environmental impact while maintaining efficiency in chemical processes. Overall, DHP is a versatile and efficient greener solvent, promoting sustainable practices in synthetic chemistry without compromising reaction efficiency. Its sustainability profile and comparable yields underline its potential for broader adoption in industrial and laboratory-scale applications.
For more information on biorenewable and greener solvents, visit The Future of Solvents: BioRenewable
References
Appendix
General Experimental Information
All commercially available reagents and solvents were used as received without further purification unless otherwise stated. Reactions were carried out under an inert atmosphere of nitrogen or argon in flame-dried or oven-dried glassware. Solvents used for reactions and chromatography were dried using standard procedures where required. Reaction progress was monitored by thin-layer chromatography on pre-coated silica gel plates (TLC aluminum sheets, Silica gel 60 F₂₅₄), and compounds were visualized using UV light or appropriate staining agents. Flash column chromatography was performed using silica gel (100–200 mesh). Organic solvents were removed under reduced pressure using a rotary evaporator, and products were dried under high vacuum. Purity and identity of compounds were confirmed by spectroscopic and analytical techniques. Nuclear magnetic resonance (NMR) spectra were recorded on a 400 MHz Bruker spectrometer. Chemical shifts (δ) are reported in parts per million (ppm) relative to the residual solvent peak. Coupling constants (J) are reported in hertz (Hz). Melting points were determined using a Buchi apparatus and are uncorrected. Specific synthetic procedures and characterization data for each compound are detailed below.
Experimental Procedures
- Synthesis of 2-(4-chlorophenyl)malononitrile (1)
A 100 mL RB flask was equipped with Dean-Stark apparatus and a thermometer pocket. To the flask was added 4-chloroacetophenone (C19708) (4.80 g, 1.0 equiv., 0.031 moles) and malononitrile (M1407) (2.46 g, 1.20 equiv., 0.037 moles) in 50 mL of DHP and stirred for 10 minutes to form a clear solution. Ammonium acetate (238074) (2.88 g, 1.20 equiv., 0.037 mmol) was added, followed by dropwise addition of acetic acid (695092) (3.0 mL). The reaction mixture was refluxed at 120 oC for 4 hours. During the reaction progress, condensed water was trapped into the Dean-Stark apparatus and removed. The reaction mixture was cooled to ambient temperature and poured into the ice-cold water. The resulting precipitate was collected by filtration and dried. The solid was recrystallized from absolute ethanol to afford 2-(4-chlorophenyl)malononitrile (1) as a pale-yellow solid (5.14 g, 82%). TLC: Rf 0.4 in 20% ethyl acetate in hexane. 1H NMR (CDCl3, 400 MHz): δ 7.51 (m, 4H), 2.65 (s, 3H) ppm. 13C NMR (CDCl3, 100 MHz): δ 173.9, 138.72, 134.16, 129.53, 128.8, 112.6, 85.17, 24.19 ppm. - Synthesis of 2-(2,6-dioxopiperidin-3-yl)-2,3-dihydro-1H-isoindole-1,3-dione (T144)
In a tubular 30 mL microwave vial equipped with a magnetic stir bar were added phthalic anhydride (320064) (1.0 g, 1.0 equiv., 6.75 mmoles) and 3-aminopiperidine-2,6-dione Hydrochloride 2 (1.21 g, 1.10 equiv., 7.43 mmoles) in a 10 mL mixture of pinane:acetic acid (8:2). To this mixture, then, sodium acetate (1.38 g, 2.5 equiv., 16.87 mmoles) was added, and the vial was closed with a pressure cap and put on the microwave reactor. The reaction mixture was irradiated at 175 oC for 2 hours while stirring at 600 rpm. The supernatant solvent was decanted, and the resulting residue was washed with ethyl acetate (50 mL). To the residue, 20 mL of water was added and stirred for 10 minutes, filtered through a Buchner funnel, and dried to afford thalidomide (T144) as a light brown solid (1.37 g, 79%). TLC: Rf 0.46 in 5% methanol: dichloromethane. The recovered DHP was recycled for further batches of reactions. 1H NMR (CDCl3, 400 MHz): δ 11.15 (brs, 1H), 7.90 (m, 4H), 5.15 (m, 1H), 2.92 (m, 1H), 2.64 (m, 2H), 2.17 (m, 1H) ppm. 13C NMR (CDCl3, 100 MHz): δ 173.8, 171.0, 170.4, 167.6, 135.4, 134.8, 133.1, 131.7, 123.9, 123.4, 49.4, 31.4, 22.5 ppm. - Synthesis of 1-chloro-2-{2-[2-(2-chloroethoxy)ethoxy]ethoxy}ethane (14625)
In a 100 mL, three-neck RB flask equipped with a nitrogen inlet and a 10 mL liquid addition funnel, tetraethyleneglycol (110175) (10.0 g, 1.0 equiv., 0.051 moles) and pyridine (270970) (37.1 mL, 3.0 equiv., 0.153 moles) were added with 10 mL of pinane. Thionyl chloride (230464) (12.9 g, 2.1 equiv., 0.108 moles) was then added to the reaction mixture through the addition funnel over the period of 10-15 minutes at room temperature (Note# effervescence was observed during the addition of thionyl chloride). The reaction mixture was cooled to 0 °C using ice-cold water, and pyridine (8.8 g, 2.2 equiv., 0.113 moles) was added carefully for 20 minutes. Then the light brown-colored reaction was allowed to stir at room temperature for 16 hours. The resulting reaction mixture was filtered off to remove the insoluble pyridinium salt by filtration. To the filtrate, then, 50 mL of absolute ethanol was added, and the light brown solution was heated at 60 °C for 3 hours. During heating, the brown solution turned into a black-colored solution. The reaction mixture was cooled to room temperature and poured over ice-cold water (50 mL) and extracted with ethyl acetate (250 mL). The organic layer was washed with saturated sodium bicarbonate (100 mL) and brine solution (100 mL), dried over sodium sulfate, and evaporated to yield crude brown to black-colored gel. Crude material was then subjected to column chromatography using 100-200 silica gel and eluted the product (50% hexane in dichloromethane to 100% dichloromethane). Pure fractions were evaporated to afford the product as colorless liquid chloride (14625) (8.7 g, 73%). TLC: Rf 0.8 in 5% methanol in dichloromethane. 1H NMR (CDCl3, 400 MHz): δ 3.77-3.74 (m, 4H), 3.67-3.61 (m, 12H) ppm. 13C NMR (CDCl3, 100 MHz): δ 71.33, 70.63, 42.76 ppm. - Synthesis of 2-(1H-imidazol-1-yl)-1-phenylethanone (3)
A solution of 2-bromoacetophenone (115835) (0.72 g, 3.6 mmol) and imidazole (I202) (0.55 g, 8.0 mmol) in dihydropinene (20 mL) in the presence of sodium bicarbonate (792519) (0.61 g, 7.2 mmol) was heated at 100°C for 8 hours. The reaction content was poured into crushed ice, the resulting solution was filtered, and the filtrate was concentrated in vacuo and purified by crystallization from ethyl acetate to obtain the phenylethanone 3 (0.35 g, 53%). TLC: Rf 0.45 in ethyl acetate in n-hexane. 1H NMR (CDCl3, 400 MHz): δ 7.96 (2H, d, J = 7.9 Hz), 7.65 (1H, t, J = 7.4 Hz), 7.57 - 7.48 (3H, m), 7.12 (1H, s), 6.94 (1H, s), 5.40 (2H, s) ppm. 13C NMR (CDCl3, 100 MHz): δ 191.7, 138.2, 134.5, 134.3, 129.6, 129.2, 128.1, 120.4, 52.6 ppm. - Synthesis of 4-(furan-2-yl)benzaldehyde (4)
100 mL, three-neck RB flask was equipped with a nitrogen inlet, a condenser, and a DHP: ethanol: water mixture (50 mL, 2:2:1) was added. The mixture was degassed using argon for 10 minutes. 4-bromo benzaldehyde (B57400) (1.0 g, 1.0 equiv., 5.4 mmoles) and 2-furan boronic acid (464910) (0.76 g, 1.25 equiv., 6.7 mmoles) were poured into the vessel and degassed for another 5 minutes. Sodium bicarbonate (792519) (0.95 g, 2.1 equiv., 11.3 mmoles) followed by tetrakis(triphenylphosphine)palladium(0) (216666) (0.31 g, 0.05 equiv., 0.27 mmoles) were added to the solution and was allowed to heat the reaction mixture at 110 oC for 12 to 14 hours. The reaction progress was monitored by TLC. The reaction mixture was cooled to room temperature and filtered through a Celite bed, diluted with ethyl acetate, and the organic layer was separated. The organic layer was dried over Na2SO4 and then evaporated to dryness under reduced pressure, followed by silica gel column chromatographic purification using 5-10% ethyl acetate in hexane, afforded benzaldehyde 4 as a colorless liquid (0.65 g, 70%). TLC: Rf 0.8 in 5% methanol in dichloromethane and Visualization in UV and 2,4-DNP charring; 1H NMR (CDCl3, 400 MHz): δ 9.99 (1H, s, CHO), 7.88 (2H, dd, J = 8.0, 2.0, H-2,6), 7.79 (2H, dd, J = 8.0, 2.5, H-3,5), 7.54 (1H, d, J = 2.0, H-5 Fur), 6.83 (1H, d, J = 3.3, H-3 Fur), 6.52 (1H, dd, J = 3.3, 2.0, H-4 Fur) ppm. 13C NMR (CDCl3, 100 MHz) δ 191.5 (CHO), 152.5 (C-2 Fur), 143.6 (C-5 Fur), 134.8 (C-4), 136.0 (C-1), 130.3 (C-2,6), 123.8 (C-3,5), 112.2 (C-4 Fur), 108.1 (C-3 Fur) ppm. - Synthesis of dibenzo(h,k)-4-oxatricyclo(5.2.2.02,6)unde c-8,10-diene-3, 5-dione (S261629, S958166)
A solution of anthracene (141062) (1.00 g, 5.61 mmol) and maleic anhydride (M188) (0.66 g, 6.73 mmol) in DHP (20 mL) was heated at 80 to 90 oC for 2 hours. During the progress of the reaction, the solution turned into a white precipitate. The reaction mixture was then cooled, filtered, and the solid obtained was washed with ethyl acetate (10 mL) and dried under reduced pressure to yield dibenzo(h,k)-4-oxatricyclo(5.2.2.02,6)undec-8,10-diene-3,5-dione (S261629, S958166) as a white solid (6.94 g, 89%). The recovered DHP was recycled for further batches of reactions. Melting point: 263°C; 1H NMR (CDCl3, 400 MHz): δ 7.40 (m, 4H), 7.24 (m, 4H), 4.87 (d, 2H, J = 1.6 Hz), 3.54 (s, 2H) ppm. - Synthesis of diethyl cyclopent-3-ene-1,1-dicarboxylate (5)
A solution of the diethyl diallylmalonate (283479) (1.00 g, 4.16 mmol) in DHP (20 mL) was degassed by bubbling argon through it. Grubbs catalyst® M204 (569747) (190 mg, 0.23 mmol) was added to the solution in portions. The resulting pink solution was stirred at 60 oC for 12 hours. The solvent was reduced under controlled vacuum, and the dark residue was purified by column chromatography to afford cyclopentene 5 as a colorless liquid (0.79 g, 90%). TLC: Rf 0.7 in 10% ethyl acetate in hexane. Visualization: UV and KMnO4. 1H NMR (CDCl3, 400 MHz): δ 5.59 (s, 2H), 4.21 (q, 4H, J = 7.7 Hz), 3.02 (m, 4H), 1.26 (t, 6 H, J = 7.16 Hz) ppm. 13C NMR (CDCl3, 100 MHz): δ 171.3, 129.2, 66.3, 52.0, 41.7, 17.1 ppm.
如要继续阅读,请登录或创建帐户。
暂无帐户?