Surface-enhanced Solar Energy Conversion Systems
Shanlin Pan, Arunava Gupta
Department of Chemistry, The University of Alabama, Tuscaloosa, Alabama 35487-0336
Material Matters, 2012 v7, n4
Solar Energy Conversion and Challenges
Sustainable, environment-friendly, and clean energy sources with sufficiently high production efficiency for practical application are highly desirable to meet the energy challenge of the 21st century due to the world′s increasing energy demand. The sun provides 1.2 × 105 TW of light for the earth and only a small fraction of the total solar energy is needed to solve the global energy problem should it be used efficiently for direct electricity generation through solar cell panels and/or clean fuel (e.g., hydrogen production through water splitting). Enormous progress has been made in recent years toward efficient and economic solar energy conversion systems. As an alternative to crystalline silicon, low-cost, stable, and high-efficiency solar cells have been developed to capture solar energy to produce electricity. Amorphous silicon has been used for solar cell panels with only limited efficiency and its production is based on energy-intensive techniques. Several types of organic photovoltaics (OPVs) have been developed as alternatives.1,2,3,4 OPVs also have limited efficiency because of the conflicting need between a thick donor layer for maximum light absorption and the bottleneck of short exciton diffusion distance.5 Poor charge-transport mobility and stability of organic semiconductors are additional factors currently limiting the overall device performance. Therefore, new solar energy conversion devices that can support efficient light absorption and hole carrier/exciton transport are highly desirable. Direct water splitting through photoelectrochemical systems continues to hold the promise of providing a practical method for producing high purity hydrogen which can be further used for energy generation, industrial processes and storage.
The ideal photocatalyst for water splitting must satisfy at least four criteria.6 First, a band gap of ~2.0 eV, which corresponds to the equilibrium potential difference between the hydrogen evolution reaction (E0=0.0 V) and the oxygen evolution reaction (E0=1.23 V) at 25 °C, and the energy losses in the semiconductor for charge carrier transport. This energy gap corresponds to a 650 nm wavelength incident light absorption. That means a photocatalyst excited with a wavelength shorter than 650 nm is needed for direct water splitting in a single-photon absorption configuration. Tandem cell and/or multiple light absorption configurations would be necessary should a wavelength longer than 650 nm be used for this photochemical reaction. Second, the valence band edge energy level of the photocatalyst (n-type semiconductor) should be lower than the H2O/O2 level, and the Fermi level should be higher than the H2/H2O level in order to obtain direct water splitting under sunlight. External bias and/or interface potential are required if one of two energy level conditions are not satisfied. Third, the photocatalysts must be stable in aqueous solution to avoid degradation that may be caused by photocorrosion. And lastly, the photocatalyst material used for direct water splitting should be inexpensive to be commercially available worldwide. Fujishima and Honda7 used TiO2 (224227) as a photoelectrode for water splitting at low efficiency, yet this semiconducting material cannot split water effectively due to its wide band gap, even though it meets all four requirements. Photocatalysts for direct water splitting that absorb in the visible spectrum have been intensely investigated since then.8 The search for more suitable semiconductors has continued, including new types of oxynitrides [e.g., (Ga1-xZnx) (N1-xOx)]9 and doped TiO2 for water splitting using visible light.10,11
Hematite (α-Fe2O3) (529311) has a favorable band gap of around 2.0 eV, is chemically stable in aqueous environments, and is one of the most abundant materials on Earth. Theoretically, a 16.8% water splitting efficiency is predicted for hematite.12 Hematite also possesses a conduction band edge at an energy level below the H2/H2O level. Thus, one needs to either apply an external electrical bias or couple the hematite electrode with a solar cell in order to enable the water splitting reaction. For example, unassisted solar hydrogen production can be achieved by incorporating hematite in a tandem cell configuration.13,14 Moreover, while hematite has a light penetration depth of 118 nm (at λ=550 nm),15 it has a very small hole diffusion length (2−4 nm).16,17 This means the majority of the photons absorbed by a relatively thick hematite layer cannot be used to oxidize water at the solid-liquid interface. This problem can be partially addressed by using nanoscale materials and doping agents. For example, silicon-doped hematite thin films and nanostructures, prepared by atmospheric pressure chemical vapor deposition (APCVD),18 can have a variety of nanostructured morphologies and exhibit a solar-to-hydrogen conversion efficiency of 3% in a tandem configuration. Thus, a different methodology is needed to further improve the overall photoelectrochemical performance of a visible-light-active photocatalyst such as hematite.
A promising technique to help improve the efficiency of photovoltaics and photocatalysts is the addition of plasmonic nanoparticles, which have been shown to enhance the efficiency of solar energy systems. This article focuses on the technical aspects of incorporating plasmonic sources of silver and gold nanoparticles into a solar cell and a water splitting system to enhance their solar energy conversion efficiencies. Characteristics of surface plasmon for light absorption enhancement are discussed first, and applications in solar energy conversion and challenges of silver and gold nanostructures in the two energy conversion systems are subsequently discussed.
Surface Plasmon Resonance of Metallic Nanostructures
Surface plasmon resonance refers to the collective oscillating motion of conduction electrons near a metal surface in an external electromagnetic field and can be excited on metallic nanoparticles, such as silver and gold. The extinction spectra of metallic nanoparticles are usually dominated by one or more well-resolved peaks, which are caused by strong light scattering and absorption. Plasmon resonance frequency is determined by the dispersion relation of the metal, particle size and shape, and the changes in dielectric constant of the surrounding medium. Coupling between individual particles and their geometry can also dramatically influence the position of the plasmon resonance.19 Surface plasmons of silver and gold nanostructures (576832 and 636347) can cause significant enhancement in the local electromagnetic field due to the intense surface plasmon resonance cross-section.20 Coupling between two or more silver (or gold) nanoparticles can have their excited states localized between the particles and produces even higher local field intensity than the individual nanoparticles.21 Such field enhancement is expected to increase the light absorption of the photoactive layers of photocatalysts for renewable energy conversion.
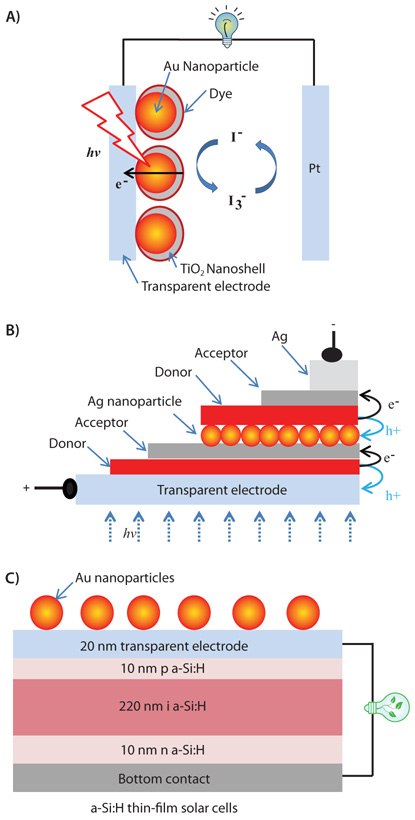
Figure 1.Schematic of surface-enhanced dye-sensitized solar cell (A), double heterojunction OPV (B), and silicon solar cell (C) with their efficiencies enhanced by gold nanoparticles.
Surface-enhanced Solar Energy Conversion Systems
Experimental evidence in the literature has shown that the power efficiency of both solar cells and photocatalytic thin-film electrodes can be enhanced in the presence of metallic nanoparticles because of their surface plasmonic effects. In the field of solar cells, gold nanoparticles have been used to enhance the efficiency of a dye-sensitized solar cell,22 an organic solar cell,23 and a silicon solar cell,24 (Figure 1.) Gold or silver nanoparticles have been incorporated into these three types of solar cells in such a way that the photoactive layers of the cells benefit from the enhanced light absorption near the plasmonic structure. For example, gold nanoparticles are embedded in a thin layer of TiO2 under the dye layer in order to enhance the absorption cross-section of the dye-sensitized solar cell (Figure 1A). The top coating of gold nanoparticles with TiO2 is critical to prevent corrosion of gold nanoparticles in the presence of liquid electrolytes. Meanwhile, the thickness control of TiO2 is very important to allow efficient field enhancement, as plasmon enhancement of the dye layer decreases when it is placed further away from the metallic surface. Recent work on surface plasmon-enhanced organic solid solar cells and relevant organic electronics have been summarized in a recent review article by Rothberg and Pan.25 Typically, efficiency of a tandem organic solid solar cell with double heterojunctions as shown in Figure 1B can be greatly enhanced by silver nanoparticles. To obtain optical light absorption enhancement, the photoactive layers of this device have to be close to the silver nanoparticle surface. Silver nanoparticles in this type of device can also function as charge recombination sites for electrons and holes from each of the two heterojunction cells to help conduct electricity through the double layer junction device. Direct surface coating with gold nanoparticles onto silicon solar cells is also found to improve the device performance by expanding its solar energy response wavelength range and light absorption cross-section (Figure 1C). Such top configuration does not interrupt the charge separation and transport of the photoactive layer in the device while enhancing its light absorption, yielding improved device performance.
In the field of direct water splitting, numerous recent studies have shown that enhanced visible light photoelectrochemical response can be observed for thin-film photoanode electrodes by incorporating silver and gold nanoparticles into the electrode (Figure 2). Yet, some studies show plasmon sources produce no effect or a decrease in photoelectrochemical efficiency. For example, Thimsen and coworkers26 show the effects of bare spherical gold particles on the photocatalytic performance of α-Fe2O3 electrodes with the gold nanoparticles embedded in the hematite layer and on its surface. The embedded gold nanoparticle configuration was found to have no effect on hematite performance; whereas the top configuration on hematite nanoplatelets showed plasmon effect on the photocurrent response. The overall power efficiency decreased in the presence of gold nanoparticles. More recently, Thomann et al.27 reported bare spherical gold nanoparticles decrease the photocatalytic behavior of α-Fe2O3 in both embedded and top configurations because gold can serve as a charge recombination center, whereas silica shell-coated gold nanoparticles can enhance photocatalytic efficiency because the charge recombination at gold nanoparticles is blocked by silica. In addition, different enhancement action spectra were observed for embedded and top configurations due to the changes in electronic structure and morphology of hematite at the solid-liquid interface in the presence of silica-coated gold nanoparticles. Using another approach, Gao and coworkers28 showed an enhanced photocurrent in a thin-film iron oxide photoanode coated on gold nanopillars. The enhancement factor from 500 nm to 700 nm, corresponding to surface plasmon absorption enhancement, is very small. Despite disagreements in the literature, new cell configurations to incorporate plasmonic nanoparticles into the catalytic layer, based on their size, shape, and surface coverage, and the thickness control of the photocatalytic layer, are critical for improved control of the surface enhancement renewable energy conversion process.
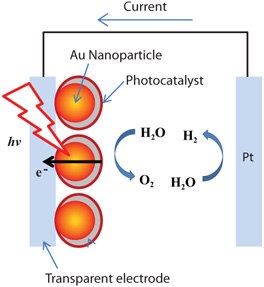
Figure 2.Surface-enhanced photoelectrochemical cell for hydrogen generation from direct water splitting using solar energy.
Conclusions
Silver and gold nanoparticles have been used in thin-film solar cells and photoelectrochemical water splitting systems to enhance their power efficiency. Wavelength dependence of photocurrent or catalytic performance should be taken into account to confirm the plasmonic effect. To avoid formation of charge traps or charge recombination sites in the presence of metallic nanoparticles, surface encapsulation is necessary. This prevents charge flow to the plasmonic antenna system when its surface enhancement capability is used to benefit the energy conversion process. Distance from the photocatalytic layer for direct water splitting or from the photoactive layer of a solar cell should be precisely controlled to optimize the surface enhancement capability. Particle size and density studies are needed to determine the wavelength range where the surface enhancement would benefit from surface plasmon enhancement.
Acknowledgment
This material is supported by the National Science Foundation under award number CHE-1153120 and the University of Alabama 2010 RGC award.
References
如要继续阅读,请登录或创建帐户。
暂无帐户?