Poly(2-Oxazoline)s: The Versatile Polymer Platform for Biomedicine
Victor R. de la Rosa1, An Van Den Bulcke2, Richard Hoogenboom1
1Supramolecular Chemistry Group, Department of Organic and Macromolecular Chemistry Ghent University, Krijgslaan 281-S4, 9000 Ghent, Belgium, 2ChemTech, Department of Organic and Macromolecular Chemistry, Ghent University Krijgslaan 281-S4, 9000 Ghent, Belgium.
Material Matters, 2016, 11.3
Introduction
The introduction of polymers into the biomedical field has opened new avenues in tissue engineering, implant design, biosensing, and drug delivery. The synergetic combination of polymers and pharmaceuticals provides a means to address significant unmet medical needs such as continuous sustained drug release, or delivery of high drug payloads to specific tissues. Thus, polymers are a key component in areas such as cancer treatment, regenerative medicine, and gene therapy.
Poly(ethylene glycol), or PEG, also known as poly(ethylene oxide), or PEO, is the most extensively used polymer in biomedicine to increase the halflife and immunogenicity of proteins. Although PEG remains the gold standard in polymer-based biomedical applications based on its low dispersity (Ð), biocompatibility, and limited recognition by the immune system (stealth behavior), it has some drawbacks and limitations. For example, anti-PEG antibodies have been observed in a significant number of patients,1,2 including 25% of patients never treated with PEG drugs (due to its ubiquity in cosmetics and food additives). This suggests the cause of the accelerated blood clearance of PEG conjugates after multiple injections.3 In addition, the polyether backbone of PEG is prone to oxidative degradation,4 a significant drawback for long-term applications such as antifouling surfaces for implants5 and probable induction of PEGmediated complement activation.6–8
Nevertheless, the success of PEG in biomedical applications has paved the way for the development of the next generation of polymeric biomaterials, with greater versatility and more diverse architectural possibilities to meet the new challenges in medicine and the requirements in drug loading, responsiveness, targeting and labeling.9–11 Poly(2-alkyl/aryl-2-oxazoline)s, commonly abbreviated as PAOx, POx, or POZ, provide higher stability, tunability, and functionalization than PEG, while retaining the requisite features of biocompatibility,12 stealth behavior, and low dispersity. The excellent properties of PAOx polymers enable their use in a wide variety of different biomedical applications, from targeted drug delivery and drug formulation to tissue engineering and tissue adhesives. In particular, the extraordinary synthetic versatility of PAOx allows the construction of complex polymeric architectures with finely tunable physical properties in a defined manner, making it an attractive platform for developing new approaches in precision medicine.13,14 This overview on biomedical applications of PAOx presents a special emphasis on their contribution and potential impact on drug delivery applications.
Properties and Biocompatibility
As shown in Figure 1, PAOx are readily obtained via cationic ringopening polymerization (CROP) of 2-oxazolines, resulting in polymers with a backbone composed of tertiary amides that suppress interactions with proteins and result in significantly reduced recognition by the immune system.15
Functionalities can be introduced at both ends of the polymer chain by selection of the electrophilic initiator [alkyl halides, acid halides, (pluri) tosylates, (pluri)triflates, (pluri)nosylates, etc.] and nucleophilic terminating agent (amines, thiolates, carboxylates, etc.). Control of the polymer chainend functionality allows incorporation of targeting units or radiolabels for imaging, while also enabling the use of PAOx for surface or nanoparticle modification. Moreover, the side chains are tunable by modification of the substituent of the 2-oxazoline monomer, granting control over the hydrophilic–hydrophobic balance and the lower critical solution temperature (LCST) of the polymer.16 This side-chain tunability enables the introduction of multiple functional groups along the polymer chain and the preparation of hydrogels or highly drug-loaded delivery vehicles.
Figure 2 shows a series of PAOx with an increasing degree of hydrophobicity. While poly(2-methyl-2-oxazoline), or PMeOx, displays a higher hydrophilicity than PEG,17 PAOx with longer alkylic side-chains exhibits a thermoresponsive behavior with transition temperatures progressively lowering with the polymer side-chain hydrophobicity. In contrast to the gold standard thermoresponsive polymer for biomedical applications, poly(N-isopropylacrylamide) (PNIPAM, LCST = 32 °C, Product No. 806471), PAOx exhibits a minimal thermal hysteresis behavior and the transition temperature can be fine-tuned by copolymerization of hydrophilic and hydrophobic 2-oxazolines.18 This tunability makes PAOx an ideal polymer for the development of stimuli-responsive smart materials, with applications in detection, diagnostics and triggered drug delivery.19–21

Figure 1. Living Cationic Ring-opening Polymerization (CROP) of 2-oxazolines. Both alpha and omega termini can be functionalized by the selection of initiator (tosylate in the scheme) and terminating agent (a nucleophile). Well-defined block copolymers are attainable by successive monomer addition, resulting in polymers with very low dispersity values (typically Ð = 1.05–1.30).
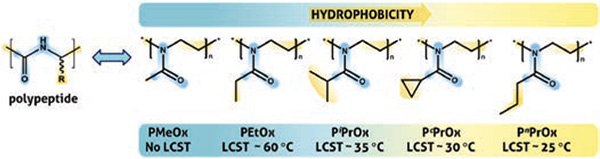
Figure 2. A series of PAOx derivatives displays the structural analogy with natural polypeptides and their amphiphilic character. The lower critical solution temperature (LCST) can be fine tuned by copolymerization. PiPrOx and PnPrOx are structural isomers and potential alternatives of poly(N-isopropylacrylamide) (PNIPAM, LCST = 32 °C).14
The structural similarity of PAOx with natural polypeptides as shown in Figure 2, accounts for their stealth behavior and excellent biocompatibility. PAOx exhibit a very fast blood clearance and remarkably low uptake in organs of the reticuloendotheliary system, as demonstrated in biodistribution studies with radio-labeled 5 kDa PMeOx and poly(2-ethyl-2-oxazoline), or PEtOx,22 that show an apparent clearance limit of 40 kDa.23 In vivo toxicity has shown no adverse effects upon repeated intravenous injections (in rats) of 10 and 20 kDa PEtOx in a broad range of concentrations (500 to 2,000 mg/kg).17 Perhaps the most reassuring instance of PAOx biocompatibility is the development of the first commercial PAOx-based pharmaceutical, which is currently undergoing first-in-human Phase I clinical trials.24
As a result of their excellent biocompatibility and synthetic versatility, PAOx are attracting a growing interest as a future platform of choice in drug delivery, and significant progress in this field has already been realized. Applications in development tackle current challenges in high drug loading targeted delivery, combination therapy, sustained drug release, and formulation. The main strategies investigated using PAOx in drug delivery are summarized in Figure 3, and can be differentiated from systems where the active pharmaceutical ingredient (API) is covalently or non-covalently linked to the polymer.
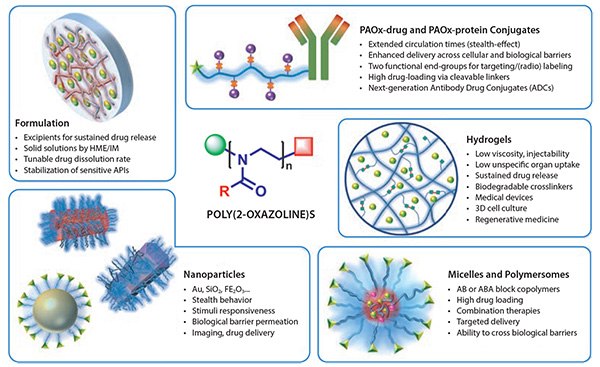
Figure 3. Overview of the main strategies that PAOx offer for drug delivery applications. The remarkable ability to tailor the material properties offered by PAOx accounts for their applicability in a diverse set of drug delivery approaches.
Non-covalently Linked Drug Delivery
PAOx as an Excipient in Drug Formulation
Perhaps the most straightforward contribution of polymers to drug delivery is their use as excipients, where the API is dispersed together with the polymer that serves as a matrix to enhance the drug solubility profile. The search for new drug excipients is motivated by the poor water solubility properties of an estimated 90% of newly developed drugs.25 Hot-melt extrusion (HME) or spray-drying of adequate polymers together with the API allows the formation of solid solutions in which the drug is stabilized in an amorphous form, highly increasing its solubility and bioavailability.
De Geest et al. prepared tablet formulations of metoprolol tartrate/PEtOx and fenofibrate/PEtOx via HME using Aquazol. For both the hydrophilic metoprolol tartrate and the hydrophobic fenofibrate, release profiles could be either accelerated or slowed down by variation of the polymer molar mass.26 Urbanova and co-workers demonstrated similar tunability of the acetylsalicylic acid release profile in solid dispersions with PEtOx.27 In addition, PAOx formulations have been shown to significantly enhance the stability of sensitive cannabinoids, performing remarkably better than state-of-the-art commercial polymers.28 Recently developed methods to produce the high molar mass, low dispersity PAOx29 required for pharmaceutical applications are expected to accelerate the use of PAOx in drug formulation.
PAOx-based Micelle Systems
Amphiphilic polymers self-assemble into micelles or polymersomes in which the morphology can be selected by tuning the polymer length and composition. Micelle systems are advantageous since they can enable high loading of drugs with poor water solubility, a challenge especially for many new cancer treatments. In addition, micelles benefit from both passive and active targeting because they tend to accumulate in cancer tissues due to the enhanced permeability and retention (EPR) effect while serving as a platform to incorporate targeting groups.
PAOx allow for highly defined polymer structure and composition enabling fine tuning of the hydrophilic–hydrophobic balance of the polymer by copolymerization and, thus, the control on micelle size and drug release properties. Most reported PAOx-based micellar systems feature a hybrid PAOx-polyester (PAOx-PE) diblock structure, or an ABA triblock structure synthesized by sequential addition of hydrophilic and hydrophobic 2-oxazoline monomers.
Zhao and co-workers used a Boc-NH-PEtOx-OH as a macroinitiator for the polymerization of ε-caprolactone and subsequently functionalized the hydrophilic PEtOx with a folate moiety. This resulted in folate-decorated micelles that could be loaded with doxorubicin (DOX, Product No. D1515) with capacities higher than 10 wt.%. These nanovectors showed better therapeutic efficacy and reduced toxicity than DOX when administered to nude mice bearing KB tumors.30 A similar targeted micelle system was loaded with indocyanine green, an FDA-approved near-infrared dye, allowing both tumor imaging as well as effective photothermal therapy of KB tumors in vivo.31
Multiple targeting moieties can be incorporated in the micelle outer shell for enhanced cellular uptake by combining differently functionalized PAOx, as recently reported for a PEtOx-b-P(d-l-lactide) system.32
A very well studied micelle system developed by Kabanov, Jordan and Luxenhofer comprises an ABA triblock structure featuring a hydrophobic middle-block of poly(2-n-butyl-2-oxazoline), or PBuOx, and two outer blocks of PMeOx.33 This polymer system yields stable micelles with sizes below 100 nm and unmatched high drug-loading capacity of anti-cancer drugs with poor water solubility. Loading capacities of up to 50 wt.% have been reported for a range of new-generation taxoids, increasing the intrinsic solubility of the APIs by up to 9,000 times.34 Synergetic effects arising from combining multiple APIs in the micelles have also been reported for these high capacity micelle systems.35 Currently, Kabanov’s team is building a cheminformatic database to predict which drugs can best take advantage of these PAOx-based micelle carriers.
PAOx-based Hydrogels
The introduction of functionality across the polymer side-chain allows a wide variety of strategies to prepare PAOx-based hydrogels.36,37 Lecommandoux et al. introduced reactive amine units along the PEtOx chain by partial hydrolysis. The obtained PEtOx–PEI copolymers were subsequently crosslinked with a bis-glycidyl ether in aqueous medium, resulting in biocompatible spherical nanogels with an optimal size for drug delivery applications.38,39 Furthermore, injectable hydrogels based on PEtOx-poly(ε-caprolactone)-PEtOx have demonstrated superior biocompatibility compared to commercial Matrigel® and Pluronic® F127 (Product No. P2443) for intraocular drug delivery in vivo.40
Dargaville et al. copolymerized MeOx and 2-(dec-9-enyl)-2-oxazoline, obtaining hydrophilic polymers with alkene side-chains that were functionalized with the CRGDSG peptide sequence to promote cell adhesion. Subsequent crosslinking in the presence of a dithiol yielded transparent hydrogels in a one-pot fashion. The mild conditions of the gelation process permitted the encapsulation of fibroblast cells during the UV-mediated curing process, obtaining three-dimensional cell-polymer constructs of interest in tissue engineering and regenerative medicine.41
Covalently Linked Drug Conjugates
PAOx-drug and PAOx-protein Conjugation or PAOxylation
The PAOxylation of a number of proteins like trypsin, catalase, serum albumin, insulin, or uricase has yielded conjugates with performances similar to their PEGylated counterparts.42,43 Interestingly, PEtOx-insulin conjugates were found to decrease blood glucose levels for up to 8 hours, four times longer than the free insulin.17 Kabanov et al. functionalized a number of piperazine-terminated PAOx with an NHS-activated ester and prepared conjugates of horseradish peroxidase.44 The protein retained 90% of its activity, and the cellular uptake was found to increase by three to six fold compared to unmodified protein when using an amphiphilic PMeOx- or PEtOx-b-PBuOx copolymer. Similar copolymers were used to conjugate superoxide dismutase 1 (SOD1), showing enhanced neuronal uptake of the conjugate in vitro and effective passage through the bloodbrain barrier in vivo.45
The introduction of clickable groups along the hydrophilic PMeOx or PEtOx chain46 has proven to be an effective strategy for protein and drug conjugation. Copolymers of MeOx and EtOx with 2-(pent-4-ynyl)-2-oxazoline, or PynOx, provided multiple linkage points for the stabilization of virus-like particles (VLP). An icosahedral VLP was formed by supramolecular assembly of 180 copies of the coat protein of bacteriophage Qβ, and its surface was decorated with azide groups using an azido-N-hydroxysuccinimide ester. Following copper-catalyzed azide-alkyne cycloaddition (CuAAC) click with PMeOx/PEtOx-ran-PynOx copolymers resulted in PAOx-wrapped VLPs with remarkably high thermal stability. Furthermore, the particle size could be controlled by the polymer length and attachment density.47
Serina Therapeutics has used similar PEtOx-ran-PynOx polymers to create a one-week long sustained release of rotigotine for the treatment of Parkinson’s disease. The API is linked to the polymer via CuAAC click chemistry using a biodegradable ester spacer, enabling sustained drug release that leads to constant plasma levels.48 This polymer, named SER‑214, is currently undergoing Phase I clinical trials and, if successful, will become the first FDA-approved PAOx therapeutic.24
Hoogenboom et al. introduced methyl ester functionalities across the polyoxazoline chain by copolymerization of EtOx with 2-methoxycarbonylethyl-2-oxazoline (MestOx).49 The authors demonstrated that the resulting methyl ester functionalities decorating the polyoxazoline chain constitute a highly versatile reactive handle, as a wide variety of moieties can be introduced by direct amidation with amines. This synthetic approach further expands the PAOx toolbox for the preparation of novel PAOx-drug conjugates.50
Future multiple drug-loaded PAOx-API conjugates will most definitely be improved by the addition of targeting moieties in the polymer chainends, such as folate groups or antibodies. There is, thus, ample room for advances in this fascinating field.
PAOx-functionalized Nanoparticles
Nanoparticles (NPs) are able to accommodate multiple functional groups while providing unique optical, electronic, or magnetic properties and, therefore, have enormous potential in biomedical sciences, including imaging and drug delivery. When connected to NPs, PAOx form a stealth corona that provides nanoparticle stabilization, prevents rapid clearance, and serves as a reliable scaffold for the incorporation of bioactive compounds. In this context, Benetti and co-workers functionalized PMeOx-OH with nitrodopamine for the functionalization of ZnO nanocrystals of interest for imaging. Functionalization with 4 kDa PMeOx yielded individually dispersed nanocrystals with an outstanding stability of up to 9 months.51
In addition to granting stability and stealth effect to NPs, PAOx thermoresponsive properties have been exploited to prepare responsive or smart NPs that aggregate upon the application of external stimuli.20 Recently, fluorescent NPs based on a polyorganosiloxane were functionalized with the thermo-responsive poly(2-isopropyl-2-oxazoline), or PiPrOx, (Figure 2). Below 31 °C, the PiPrOx nanoparticles exhibit an anti-fouling behavior when dispersed in a serum-containing medium. However, heating beyond this temperature triggers the adsorption of serum proteins on the nanoparticles, reversible by lowering the temperature. This strategy could be applied to increase nanoparticle agglomeration in the targeted cells or organs by applying local heating.19
Finally, as seen before for PAOx-based micelles and conjugates, PAOx can enhance nanoparticle permeation through biological barriers. Khutoryanskiy et al. used 5 kDa alkyne-terminated PEtOx to functionalize thiolated silica NPs via thiol-ene chemistry. The resulting NPs exhibited enhanced permeation through porcine gastric mucosa ex vivo in a similar way as analogous PEGylated NPs.52 Considering the straightforward tunability of PAOx composition and hydrophilic–hydrophobic balance, further developments are expected to bring even more efficient drug delivery vehicles with improved ability to permeate biological barriers.
Conclusions and Outlook
The large number of possibilities offered by polyoxazolines for creating materials that impact a broad variety of biomedical applications is unique for this polymer class. PAOx have already demonstrated excellent biocompatibility and stability in a large number of independent studies, and the first in-human Phase I clinical trials of a polyoxazoline therapeutical are ongoing. By virtue of their structural and functional diversity, and the high control and definition that the polymerization of oxazolines offers, PAOx constitute a very attractive and versatile platform for the development of next-generation drug delivery systems.
Acknowledgments
V.R.R. would like to thank Flanders Innovation and Entrepreneurship for financial support.
References
如要继续阅读,请登录或创建帐户。
暂无帐户?