Molecular Monolayers on Silicon Surfaces
Gregory P. Lopinski, Daniel D.M. Wayner
Steacie Institute for Molecular Sciences, 100 Sussex Dr., Ottawa, Ontario, Canada
Introduction
Controlled formation of organic molecular monolayers on silicon surfaces offers the promise of enhancing the functionality of existing and emerging silicon based materials and devices. The synthetic tunability and diversity of properties of organic molecules suggests a range of promising applications for the resulting hybrid organic/silicon structures. At the simplest level, molecular monolayers can serve to passivate the surface, protecting the underlying substrate or structure from unwanted reactions or processes which degrade its properties. However, these monolayers can be much more than passive protective coatings, controllably altering the properties and imparting new functionality to bulk or nanostructured materials. Formation of monolayers with a variety of terminal functional groups can be used, for example, to tailor the wettability of the surface or modulate the electronic properties of the substrate through long-range field effects. Immobilization of biomolecules or other types of selective receptors on the surface offers opportunities for the development of novel sensing platforms based on electrical, optical or mechanical transduction of chemical binding events. Attachment of redox active or other types of “molecular switch” molecules (e.g., photochromic or electrochomic molecules) to silicon could enable fabrication of molecular scale memory or logic elements integrated with conventional silicon based microelectronic devices.
This article briefly reviews the methods and mechanisms for the formation of molecular monolayers on silicon surfaces, the properties of these monolayers and current perspectives regarding their application in molecular electronic and sensing applications.
Monolayer Formation — Methods, Mechanisms and Properties
In 1993, Linford and Chidsey reported the formation of monolayers of long chain alkyl molecules covalently attached directly to silicon surfaces, without an intervening oxide layer.1 This approach involved the reaction of free radicals, formed by the thermal decomposition of diacyl peroxides, with the atomically flat hydrogen terminated Si(111) surface (H-Si(111)). The H-Si(111) surface can be generated by a straightforward wet chemical etching procedure in ammonium fluoride2 and exhibits sufficient stability to permit some degree of manipulation in air and organic solvents. Numerous subsequent studies of the reactivity of H-terminated silicon have led to the development of a range of solution and gas phase approaches to covalently attach organic molecules to the silicon surface. It is now possible to form monolayers with a wide range of terminal functionality as well as to vary the nature of the linking group (Si-C, Si-O, Si-N)3,4. These chemistries have also been employed to functionalize other H-terminated silicon substrates including Si(100) (the substrate most commonly used in the microelectronics industry) as well as porous silicon, nanowires and nanoparticles.
Following the initial reports of monolayer formation initiated by thermal decomposition of peroxy compounds, subsequent investigations revealed that terminal alkenes react under both thermal5 and photochemical conditions6 with H-terminated silicon, without the need for a radical initiator. These reactions have been shown to proceed via the radical chain reaction mechanism (Figure 1). Once an initial Si radical (dangling bond) is formed alkenes react readily at this site, by forming a Si-C covalent bond, breaking the carbon-carbon double bond and creating a carbon-centered radical. This radical can then abstract a hydrogen atom from an adjacent silicon (effectively, a [1,5] hydrogen atom shift) creating a new reactive silicon-dangling bond, continuing the process. Scanning tunnelling microscopy images of partially reacted surfaces (Figure 1) reveal that the reaction does indeed proceed via the formation of irregularly shaped islands, consistent with a pseudo-random walk like progress of the chain reaction.7,8 While the reactions propagate via a radical chain reaction, the mechanism of formation of the initial Si radical is a matter of considerable debate. Direct photochemical or thermal induced cleavage of the Si-H bond requires photons at wavelengths < 160 nm or temperatures in excess of 300 °C, whereas typical conditions for the solution phase hydrosilylation reactions involve ~300nm photons and temperatures in the range of 100–160 °C. Studies of gas phase photochemical reactions of alkenes with Si-H (using 185 nm irradiation) have implicated alkyl radicals produced via photolysis of the alkenes as the initiating species.9 In the case of thermal reactions trace oxygen is a possible initiator. The abstraction of H by molecular oxygen to create a silyl radical has indeed been observed in the molecule tris(trimethylsilyl)silane (Product No. 360716),10 an interesting molecular analog of the H-Si(111) surface. The activation barrier for this process has been calculated to be ~130 kJ/mol,11 implying that this pathway can account for reactions observed at temperatures >100 °C. The mechanism of the solution phase photochemical reactions (particularly those initiated by visible light) remains an open question.
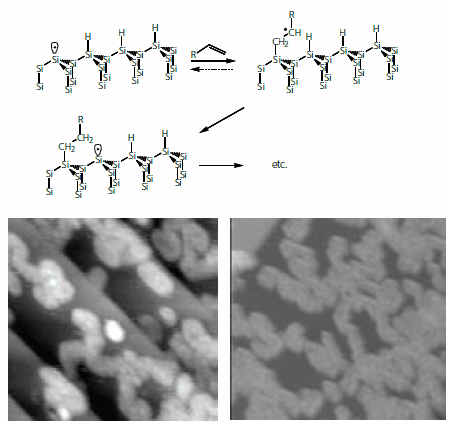
Figure 1.The reaction of alkenes with H-Si(111) proceeds via the radical chain reaction first suggested by Chidsey and co-workers5 and depicted schematically above. STM images of partially reacted monolayers show that monolayer growth proceeds via growth of irregularly shaped islands arising from the pseudo random walk progress of this chain reaction. Similar morphologies are observed for reaction in solution8 (image at right, 30 min. reaction with decene at 447 nm irradiation) or gas phase9 (image at left, 3 min. reaction with hexene at 185 nm irradiation).
Molecular monolayers made via reactions of alkenes with H-Si(111) are often referred to as “self-assembled” monolayers on silicon surfaces by analogy with thiol monolayers on gold. However, STM imaging shows that the monolayers on silicon do not exhibit the ordered domains observed for thiol-based monolayers on gold surfaces. The lack of order arises from three factors: 1) formation of strong Si-C covalent bonds precludes diffusion of the molecules once bound to the surface, 2) difference in the spacing between Si atoms on Si(111) (0.385 nm) and the diameter of an alkyl chain (0.42 nm) implies that not all Si atoms can bind an alkyl chain (cf., the 0.5 nm spacing on Au(111) for the typical √3R30 structures), and 3) the self-limiting pseudo random- walk progress of the radical chain reaction. While the last factor is particular to modification reactions that proceed via the radical chain process, the first two factors will limit the degree of order for alkyl monolayers formed via other routes such as reactions with alkyl Grignards12 or diazonium compounds.13
The density (saturation coverage) of these monolayers has been the subject of some debate. Due to the steric constraint noted above, only methyl groups can occupy all the available silicon sites (i.e., form a complete monolayer). For longer alkyl chains (8–18 methylene groups), experimental estimates of coverage generally fall in the range of 0.30–0.45 mL (i.e., replacement of 30–45% of the initial Si-H bonds with alkyl chains).7 A coverage of 0.4 mL on Si(111) corresponds to an area per molecule of 32 Å.2 This is considerably less than the density of ~21 Å2 that can be achieved for SAMs on gold substrates or in close packed Langmuir-Blodgett films.
These monolayers are chemically quite robust, withstanding boiling and sonication in various solvents (water, chloroform, aq. HCl, etc.). Monolayers formed via Si-C links are generally stable upon dipping in hydrofluoric acid, although this procedure does remove alkyl chains attached via Si-O-C bonds. While the alkyl monolayers themselves are quite stable, degradation of the underlying silicon substrate has received less attention, particularly in view of the fact that silicon surfaces are known to be highly susceptible to oxidation. X-ray photoelectron (XPS) spectra of even carefully prepared surfaces invariably show a significant O1s core level signal, corresponding to 0.1–0.5 mL of oxygen,7 likely arising from oxygen insertion into Si-Si backbonds. The absence of a shifted Si2p core level at ~103eV is often cited as an indication of an absence of silicon oxidation. However, this feature is associated with formation of SiO2 (Si in a +4 oxidation state) indicative of the later stages of oxidation. In contrast, insertion of an oxygen atom into one out of the three available backbonds for each Si atom (i.e., 1 mL of oxygen) will only shift the Si2p level by ~1eV which would be difficult to resolve in most reported XPS spectra. As even low levels of oxidation can give rise to electrically active defects (see below), the reduction of oxygen concentrations in these monolayers remains a challenge. In terms of longer-term stability, alkyl termination does significantly slow down the oxidation relative to the unpassivated H-terminated surface although some level of oxidation is still observed, particularly in aqueous environments. This is not surprising in view of the fact that the monolayer is disordered and not close packed, but suggests a possible problem for using these monolayers for biosensor applications requiring extended immersion in aqueous buffer solutions.
Functional Monolayers and Biomolecule Immobilization
While methyl terminated monolayers can be useful for passivation and chemical stabilization, the low reactivity of this terminal group makes further manipulation of the surface physical or chemical properties difficult. In order to incorporate more complex organic or bioorganic structures at the interface, new strategies for coupling these molecules to the surface are required. A common approach involves the reaction of bifunctional molecules with the surface, with one end binding to the surface and the other terminal group available for further reaction. However, care must be taken to avoid both terminal groups reacting with the surface or formation of monolayers with mixed termination. In many cases it is necessary to protect one of the terminal groups in order to obtain the desired functionality. For example, amino-terminated monolayers are useful for the binding of biologically relevant molecules such as DNA and proteins. However, since the amine group can react directly with the H-terminated surface, particularly under UV irradiation, it must be protected. A common protecting group is tert-butoxycarbonyl (t-Boc), which can be removed by treatment with trifluoroacetic acid (TFA, Product No. 299537). Amine terminated monolayers made by this route have been used to attach thiol-modified DNA oligomers to the silicon surface using a heterobifunctional cross-linker.14
On the other hand, alkene esters, acids and epoxides do not require protection as they appear to react primarily via the alkene end, leaving the terminal groups available for further reaction.15–18 The observation that undecylenic acid (Product No. 124672) reacts preferentially at the alkene end, was somewhat unexpected as the oxophilic nature of silicon should thermodynamically favor reaction with the carboxylic acid group.18 The preferential reactivity with the alkenyl end is consistent with a free radical, rather than a nucleophilic mechanism. The acid function can be activated with N-hydroxysuccinimide (NHS, Product No. 130672) (Figure 2) to facilitate coupling with amine-tagged molecules. This strategy has been used to attach oligonucleotides as well as methoxytetraethylenegycol (TEG) (a compound known to inhibit non-specific binding of biomolecules to surfaces).18 FTIR is a particularly effective probe of this reaction sequence (Figure 2). The carbonylstretching mode is observed to undergo characteristic changes upon NHS activation from a single adsorption at 1715 cm–1 characteristic of a free acid to peaks at 1815 cm–1, 1787 cm–1 and 1744 cm–1 assigned to the succinimidyl ester. Upon reaction with (TEG) amine the NHS peaks are seen to disappear, replaced by new peaks at 1650 cm–1 and 1550 cm–1 assigned to the carbonyl and CNH vibrations of the amide.
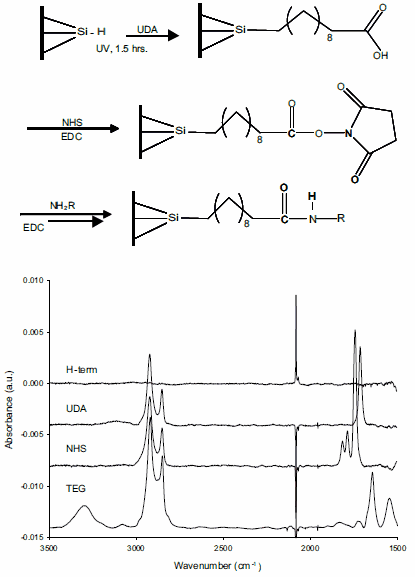
Figure 2.General multi-step reaction scheme for covalent attachment of amine tagged molecules to the silicon surface.18 The baseline corrected FTIR spectra, obtained in the attenuated total reflection (ATR) geometry, illustrates the changes in the vibrational modes of the monolayer upon passing through the various steps; H-termination followed by photochemical reaction with undecylenic acid (UDA), NHS activation and reaction with tetraethylene glycol (TEG) amine.
For the attachment of biomolecules or other larger complex molecules it is useful to have the ability to control the density of reactive sites since the molecule to be immobilized typically exhibits a much larger footprint than an alkyl chain. This has been demonstrated in the case of reactions of alkene esters16 where the density of reactive groups was controlled simply by diluting the “active” molecule in a solution of 1-alkene. While the incorporation of the reactive group has been shown to approximately correspond to the ratio of the two alkenes, whether these reactive groups are randomly distributed or clustered remains an open question.
Electronic Properties
A primary motivation for using silicon as a substrate for forming molecular monolayers involves exploiting the long-range electric field effects induced by binding events or reactions that involve charge re-distribution at the interface. These field effects arise because, in contrast to metals, electric fields penetrate substantial distances into semiconductors, shifting energy levels in the near surface region. Adsorption of charged or polar molecules on the surface is expected to shift the electronic states (a process referred to as band-bending), altering the conductivity of the substrate, in a manner analogous to the use of an external electric field to control conductivity in a field effect transistor (MOSFET). In principle, this effect can be utilized for label-free detection of chemical or biological species.
Hydrogen terminated silicon surfaces are ideal systems for probing field effects induced by adsorption and reaction events because these interfaces exhibit a low density of electrically active surface states.19 Surface states are allowed energy levels created in the normally forbidden gap region of a semiconductor which can act as traps for free carriers, reducing the sensitivity of the substrate to external fields. For molecular monolayers to be useful in electrical sensing applications the creation of these electrically active surface states must be minimized during their preparation (i.e., they should maintain the low density of these states observed on H-terminated surfaces). A further requirement for biosensing applications is that this low density of surface states must be maintained upon prolonged exposure to aqueous buffers.
Surface photovoltage (SPV) measurements using a Kelvin probe offer a contactless approach to determining band bending due to surface charges.20 Since photogenerated electron hole pairs act to screen the surface charge responsible for any band-bending, flattening the bands (provided the light intensity is high enough), the difference in surface potential in the dark and under illumination corresponds to the amount of band-bending. H-terminated surfaces typically show a small (<20 mV) photovoltage (Figure 3), which for the low doped wafers (~1x1015 cm–3) used here corresponds to a level of electrically active defects of ~1x1010 cm–2 as expected. Upon formation of an alkyl monolayer the SPV is typically seen to increase slightly to 60–100 mV. This small level of band-bending corresponds to a density of trapped charges of <5x1010 cm–2 which likely arise from unwanted oxidation of the interface during the functionalization reaction. The SPV can be reduced to close to that of the initial H-terminated surface by a brief HF dip (which cleaves Si-O-Si bonds due to oxygen insertion in the backbonds but leaves the monolayer intact as discussed previously). In order to probe the stability of these surfaces, growth of the SPV has been studied upon exposure to phosphate-buffered saline (PBS) solution. The SPV is observed to increase significantly after 1 hour in the PBS solution (Figure 3). The growth of electrically active defects in PBS has also been noted in electrochemical impedance studies of these surfaces.21

Figure 3.Surface photovoltages for different alkyl monolayers on Si(111). At the top left, the changes in SPV are shown upon formation of a decyl monolayer via photochemical reaction and subsequent exposure to PBS buffer solution followed by an HF dip. The growth of the SPV indicates an increase in the amount of trapped charge at the interface. In contrast, as shown in the bar graph on the right, a branched surface (Gen 1) formed by the reaction of pentyl Grignard with an undecylenic ethyl ester monolayer (UDE) is seen to maintain a stable and low SPV even for extended exposure to PBS buffer.
In order to improve the ability of these monolayers to maintain a low density of electrically active defects in aqueous solutions we have developed a strategy to effectively double the density of alkyl chains on the surface via a branching reaction. As noted above, prepared monolayers on silicon have densities that are typically only 50% of that of a close packed alkyl monolayer. Ester-terminated monolayers, however, can react with alkyl Grignards to create the branched structures (Figure 3), leading to an effective coverage of alkyl chains of ~0.8 mL. When the stability of these surfaces is tested the SPV remains low even for prolonged exposures to PBS.
Applications of Molecular Monolayers on Silicon
The progress in developing strategies for the controlled formation of functional monolayers and understanding the properties of the resulting silicon/organic structures has opened up a range of opportunities for designing and fabricating hybrid electronic devices and sensors based on these monolayers. Using the various functionalization strategies outlined above it is now possible to modify a wide range of silicon based structures and devices. Demonstrations of the utility of these monolayers in diverse applications such as molecular electronics and chemical/biomolecular sensing are growing rapidly. For example, monolayers of redox active porphyrin molecules attached to silicon have demonstrated charge storage characteristics that appear promising for molecular scale memory applications.22 In terms of sensing, Si microcantilevers have been modified by robust monolayers with quarternary ammonium termination to sense chromate ions in solution.23 Oligonucleotide hybridization has been detected on functionalized H-terminated surfaces of both Si(111)24 and silicon nanowires,25 demonstrating the potential of these monolayers as platforms for label-free electrical detection of biomolecules.
In summary, the future for molecular monolayers on silicon surfaces, nanostructures and devices appears to be full of possibilities, both for fundamental scientific studies and practical applications. While much progress has been achieved, many open questions remain. We expect that this field will continue to be a vibrant one, continuing to illustrate the potential made possible by the convergence of surface science and organic chemistry.
References
如要继续阅读,请登录或创建帐户。
暂无帐户?