Photo-Crosslinkable Gelatin Hydrogel: Versatile Materials for (High Resolution) Additive Manufacturing
Jasper Van Hoorick1, 2, Aleksandr Ovsianikov3, 4, Peter Dubruel1, Sandra Van Vlierberghe1, 2
1Polymer Chemistry and Biomaterials Group, Centre of Macromolecular Chemistry, Ghent University, Krijgslaan 281, S4, 9000 Ghent, Belgium (www.pbm.ugent.be), 2Brussels Photonics, Department of Applied Physics and Photonics, Flanders Make and Vrije Universiteit Brussel, Pleinlaan 2, 1050 Elsene, Belgium (www.b-phot.org), 3Institute of Materials Science and Technology Technische Universität Wien, Getreidemarkt 9 1060 Vienna, Austria, 4Austrian Cluster for Tissue Regeneration (www.tissue-regeneration.at), 5Department of Materials Science and Engineering, The University of Texas at Dallas, Richardson, TX 75080, USA
Material Matters, 2018, 13.3
Introduction
Gelatin has long been of interest to those in the field of tissue engineering. Gelatin is derived from collagen, the main constituent of the natural extracellular matrix (ECM) and interacts with cells through the arginine-glutamine-aspartic acid (R-G-D) sequences in its protein backbone, while also being enzymatically degradable.1 Gelatin is produced as a by-product of meat production, rendering it extremely cost-effective,2 and it is considered safe by the Food and Drug Association (FDA) due to its long track record in the food and pharmaceutical industries.3
Gelatin has an upper critical solution temperature (UCST) of around 30–35 °C, meaning it is water soluble above this temperature and forms a hydrogel at lower temperatures.4–6 This temperature-dependent transformation is useful for extrusionbased rapid manufacturing (RM) technologies, as a shape introduced by extrusion from a heated nozzle can be locked in by using a cooler environment.7
However, this property also means that the material does not remain stable in physiological or cell culture conditions. As a result, gelatin was originally used only as a temporary cell carrier, enabling a more straightforward manipulation of cells, or it was stabilised by coupling the primary amines present in the (hydroxy)lysine and ornithine functionalities to the carboxylic acids in aspartic and glutamic acid (resulting in a crosslinked hydrogel network), or by crosslinking nucleophilic functionalities using gluteraldehyde. Either of these procedures limited control over the design of 3D structures.3,8,9
Fortunately, gelatin stabilization strategies took a giant leap forward in 2000 when our research group developed and patented gelatin-methacrylamide (Gel-MOD), the first photocrosslinkable gelatin derivative, thereby enabling convenient and straightforward material processing.6,10 Functionalization occurs through the reaction between primary amines present in the side chains of the (hydroxy)lysine and ornithine in gelatin and methacrylic anhydride, which introduces methacrylamides.6 Since its introduction, this gelatin-methacrylamide has been applied to a plethora of biofabrication strategies, and is considered a gold standard in the field.4,7,11–17 This material, discovered in academia, is now offered commercially by several companies as a bioink for biofabrication purposes.18–20
Subsequently, several other photo-crosslinkable gelatin derivatives suitable for biofabrication (Figure 1) have emerged. They can be subdivided into two classes based on the applied crosslinking mechanism of either chain-growth or step-growth polymerization. This article focuses on photo-crosslinkable derivatives for biofabrication purposes that have application in high-resolution additive manufacturing.
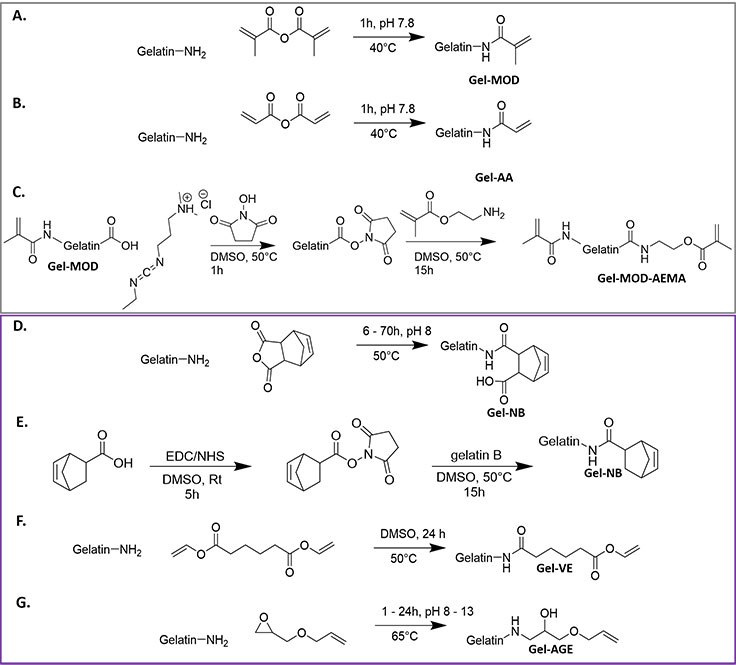
Figure 1. Overview of preparation methods of different photo-crosslinkable gelatins: chain-growth derivatives (grey, upper frame): A) gelatinmethacrylamide (Gel-MOD),6 B) gelatin-acrylamide (Gel-AA),21 C) Gel-MOD-AEMA,5 step-growth derivatives (purple, lower frame), D) gel-norbornene (Gel-NB) synthesized via reaction with carbic anhydride,1 E) Gel-norbornene (gel-NB) synthesized via reaction with 5-norbornene-2-carboxylic acid,22 F) gel-vinyl ester (Gel-VE),23 G) allylated gelatin (Gel-AGE).24
Crosslinking mechanisms
Chain-growth crosslinking
Most crosslinkable gelatins are formed using a chain growth crosslinking approach. In this mechanism, the reactive functionalities (typically (meth)acrylates or (meth)acrylamides) immobilized on the gelatin chains are polymerized with each other, resulting in the formation of short oligomer/polymer chains between the gelatin chains (Figures 2A and C).5,6,11,21,22 This enables straightforward material handling, with only material dissolution and addition of a suitable photo-initiator prior to crosslinking. No crosslinking agent is required. These solutions remain stable longer above the UCST than thiolene based systems that have a half-life ranging from 11 h at 0 °C to only 0.2 h at 40 °C (discussed below). Gel-MOD, for example, can be kept at 40 °C for over 24 hours without any problems. This level of stability is typically required during additive manufacturing processes or for cell encapsulation experiments.5,25 Moreover, chain growth provides stiffer hydrogels (Figure 3).22 A drawback of chain-growth crosslinking is the formation of a more heterogeneous network, which is prone to more shrinkage during crosslinking. Furthermore, the kinetic profile of free radical chain-growth polymerizations is usually more complicated because of diffusion limitations, chain-length issues, and reaction diffusion limitations to termination, leading to a lower degree of control over the number of reacted functional groups.26,27 The crosslinking reaction is also prone to oxygen inhibition, which is a problem for use in cell encapsulation experiments and influences reaction reproducibility. Finally, a higher spatiotemporal energy is required to crosslink gelatin compared to the thiol-ene-based step-growth hydrogels discussed below.22
Step-growth crosslinking
The second type of photo-crosslinkable hydrogels uses a stepgrowth polymerisation approach to introduce crosslinks into the hydrogel. A step-growth mechanism occurs between two complementary reactive groups, which can ideally only react with each another.27 The most common crosslinker uses thiolene “photo”-click chemistry (Figure 2B), which can form a network of any thiol with any ‘ene’ functionality either following a light-induced, radical-mediated thiol-ene reaction, or by the formation of an anionic species resulting in a thiol Michael-type addition.27 The light-induced reaction usually proceeds via the formation of a thiol-based radical which can be generated by irradiation either in the presence or in the absence of a photoinitiator, followed by reaction with the double bond of the ‘ene’ species (Figure 2B).27 In general, the reaction proceeds well with any type of non-sterically hindered ‘ene’ functionality, but an ‘ene’ functionality that cannot undergo competitive chain-growth homo-polymerization (such as norbornenes and vinyl ethers)27 is preferred. This also provides superior control over the reaction and concomitant homogeneity within the resulting network.27 To obtain a thiol-ene photo-crosslinkable gelatin, it has to contain ‘ene’ functionalities (typically norbornene, vinyl esthers or allyl ethers) which can be crosslinked using a multi-dentate, thiolated crosslinker (e.g. dithiothreitol) (Figure 2C).1,22–24 Alternatively, gelatin can be functionalised with thiols and then crosslinked using a multi-dentate ‘ene’ crosslinker (e.g. polyethylene glycoldiacrylate PEGDA).28,29
Thiol-ene ‘photo-click’ hydrogels form more homogeneous networks with less shrinkage than chain-growth hydrogels due to the highly orthogonal nature of the reaction.30 Additionally, they are not susceptible to oxygen inhibition and generally have faster reaction rates (e.g. gel-point for Gel-NB + dithiothreitol (DTT) 2.7 s vs Gel-MOD 64.7 s).22,27,30 The reaction rate is highest when norbornene functionalities are used, due to their “spring-loaded” behavior that results from the ring strain relief upon reaction, as well as the rapid thiol-hydrogen abstraction rate by the carboncentred radical.1,24,31 Furthermore, the percentage of reactive functional groups can be fully controlled by varying the thiol-ene ratio prior to crosslinking.22,27,30 In general, thiol-ene systems are more suitable for cell encapsulation than chain-growth systems, since the concentration of radical species is generally at least one order of magnitude lower than that of chain-growth hydrogel systems (where more radicals need to be formed to overcome oxygen inhibition).1,30,32 Drawbacks of the step-growth method include the necessity of an additional multi-dentate, thiolated crosslinker in the reaction mixture, which cross-reacts with other thiols to form disulfides.26 The probability of disulfide formation increases over time at elevated temperature (e.g. the half-life of DTT at pH 8.5 shifts from 11 h at 0 °C to only 0.2 h at 40 °C). This complicates the issue significantly, since higher temperatures are essential to maintain gelatin solubility during the bioprinting process.25 Furthermore, step-growth hydrogels generally have significantly lower storage moduli than their chain-growth counterparts.1,22
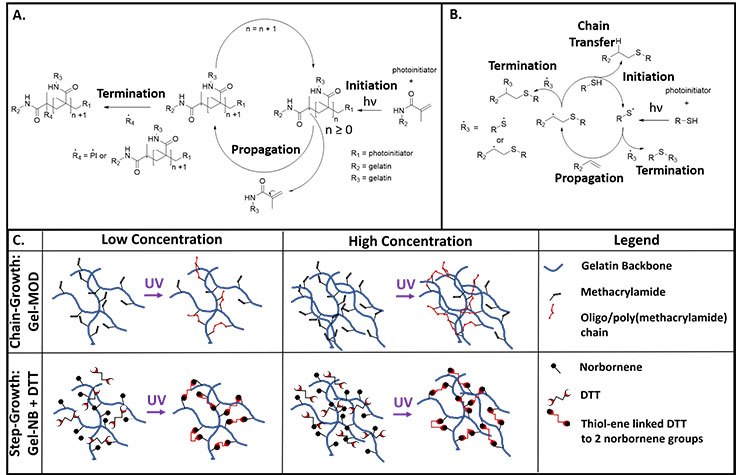
Figure 2. Illustration of chain-growth (Gel-MOD) vs step-growth (Gel-NB + DTT) crosslinking using thiol-ene photoclick chemistry. Adapted with permission from reference 22, copyright 2018 Wiley.
Controlling mechanical properties of photocrosslinkable gelatins
The mechanical properties of photo-crosslinkable gelatin hydrogels can be tuned by adjusting various parameters of the gelatin itself or during material processing.
Influencing the mechanical properties by chemical modification
The number of crosslinkable functionalities has a large effect on the final mechanical properties of the material.4,5 For most derivatives, the number of reacted primary amines, and thus the degree of substitution, can be controlled by varying the molar ratio of the functionalizing reagent (e.g. methacrylic anhydride,4 carbic anhydride,1 and 5-norbornene-2-carboxylic acid22) to match the number of primary amines present in the gelatin. When all primary amines are converted into crosslinkable functionalities, the mechanical properties can be increased even further by modifying the carboxylic acids in the side chains of aspartic acid and glutamic acid with additional crosslinkable functionalities such as 2-aminoethyl methacrylate.5 As a consequence, up to 5 times stiffer hydrogels can be obtained.5 Alternatively, the mechanical properties of gelatin-methacrylamide can be altered through covalent linking to a biopolymer such as alginate prior to crosslinking.33 Although this resulted in a weaker hydrogel blend than gelatin-methacrylamide, the modification enabled fine control of the final mechanical properties through incorporation of divalent cations to physically crosslink the alginate chains.33 In addition, the protein and polysaccharide chains formed a crosslinked network, resulting in a hydrogel that more accurately mimics the ECM.33
Influencing the mechanical properties during hydrogel processing
Once the crosslinked gelatin hydrogel has been synthesized, there are several ways to influence its mechanical properties during processing. For example, one approach is to co-crosslink it with another photo-crosslinkable material, which can be either natural (e.g. polysaccharide) or synthetic (e.g. PEG).1,34,35
However, if a single material type is required, the mechanical properties of the final hydrogel can be influenced by varying the gelatin concentration in the hydrogel precursor solution. The higher the initial gelatin concentration, the stiffer the resulting hydrogels created.4,5,21,22 However, evidence suggests that high gelatin concentrations (> 15 w/v%) also negatively affect biocompatibility.21
Variation in the amount of irradiation applied during crosslinking can also affect the final mechanical properties of the material.5–7,21 Generally, lower doses result in lower crosslink densities and thus weaker hydrogels,7,21 but lower doses also yield more unreacted, potentially cytotoxic functionalities. Additionally, when chaingrowth hydrogels are used, lower irradiation doses often reduce reproducibility due to the complex reaction kinetic profile and oxygen inhibition during crosslinking.27 Furthermore, when using highly reactive thiol-ene systems, the influence of the dose is less apparent since the material can fully crosslink at very low doses, though there is a clear correlation between irradiation energy and swelling degree. For example, during two-photon polymerization, 20 mW irradiation at 100 mm/s results in a fully crosslinked network from 40 mW onwards when applied to gel-NB, but more than 80 mW is required to fully crosslink gel-MOD.5,22

Figure 3. Scheme of the mechanical properties of different crosslinked gelatin hydrogels compared to the mechanical properties of various tissue types. Adapted with permission from reference 5, copyright 2017 ACS, with the inclusion of the mechanical properties of thiol-ene hydrogels from references 22 and 24. Hydrogels presented are depicted in red, native tissues are depicted in black, chain-growth hydrogel systems are depicted in grey, and stepgrowth hydrogel systems are depicted in purple.)
When using thiol-ene hydrogel systems, additional controls are possible. Varying the thiol-ene ratio or the amount of applied crosslinker allows fine control over the number of reacted functionalities, as well as the mechanical properties of the final product.1,22,27 A higher number of reactive thiols per crosslinker molecule results in stiffer hydrogels, as the crosslink density increases.1
This flexibility allows production of gelatin hydrogels suitable for a broad range of mechanical properties. A non-exhaustive overview of the mechanical range of gelatin derivatives compared to the mechanical properties of different tissues can be found in
Figure 3.5 As a consequence, gelatin-based materials are extremely versatile tools for mimicking the mechanical properties a plethora of tissues.
Gelatin processing via additive manufacturing
Gelatin hydrogels have been processed using a wide range of additive manufacturing techniques, including both direct methods, in which the material is applied directly in the additive manufacturing (AM) step,7 and indirect methods, where a template generated using AM is applied to control the shape of a secondary hydrogel material. Indirect approaches are usually applied to combine the mechanical properties of a stiff polymer with the desirable cell interactivity of gelatin, or to introduce complex 3D structures into materials which cannot be processed through a direct AM method.4,12,15,36
Here, we focus on light-induced additive manufacturing techniques, more specifically, high-resolution additive manufacturing techniques such as two-photon polymerization (2PP), which is often referred to as direct laser writing. The technique uses two-photon absorption to induce localized crosslinking, resulting in sub-micrometer spatial resolution. By tightly focussing a femtosecond laser beam into the material, conditions can be met for simultaneous interaction of two photons with half the energy required to bridge the band gap required for photoinitiator excitation (Figure 4A), resulting in highly localized polymerization.5,37,38 Furthermore, since the probability of 2PP is only high in the very small voxel determined by the applied optics and laser power, the polymerisation is confined to a 3D volume element often smaller than the expected diffraction limit. This is in contrast to conventional light-based additive manufacturing techniques using single photon (i.e. linear) absorption, where polymerization can occur throughout the entire beam path and is only limited by its penetration depth.5,39
As a consequence, structures with subcellular dimensions can be produced with 2PP, making it suitable for studying more complex cell-biomaterial interactions. In 2011, we were the first to report the generation of gelatin-based tissue engineering scaffolds using primary adipose tissue-derived stem cells using this method.14
Since then, multiple studies have been reported dealing with 2PP processing of modified gelatins, most of them to date using gelatin-methacrylamide (Gel-MOD).23,40,41 Using this gelatin derivative, the successful use of 2PP was even reported in the presence of living cells.13 Although the cells did not survive direct exposure to the laser during structuring, it was possible to use 2PP to entrap cells within 3D microstructures (Figure 4B).13 Furthermore, cytotoxicity was not due to spatiotemporal irradiation by the laser or the photo-initiator, but instead could be attributed to formation of cytotoxic species within the cells as a side-product of photo-initiator activation.13 This was later substantiated with a macromolecular photo-initiator based on hyaluronic acid, which enabled 2PP processing combined with the encapsulation of living cells, even within the exposed areas (Figure 4C).42 The study indicated that the previously observed cytotoxicity originated from the penetration of the low molecular weight photo-initiator through the cell membrane, thereby resulting in photo-oxidative damage within the cell during irradiation. By immobilizing the photo-initiator onto a macromolecule, cell barrier penetration was prevented, thereby allowing 2PP in the presence of living cells.42
Despite these successful approaches, gelatin-methacrylamide does have some limitations for 2PP processing. In general, due to poor reaction kinetics and associated mechanical properties, relatively high gelatin concentrations (>15 wt%) and light dose (eg. 330 mW at 7 mm/s scan speed) are required to crosslink the material.13,14,40,43 Most importantly, subsequent swelling of the 2PP-produced structures can negate the high-resolution capacity of this approach.
Other strategies involve the application of a secondary material to function as a mechanical support,35,43 either by indirect additive manufacturing (discussed below), or by first structuring a stronger material to function as support, such as a mixture of hydrophobic acrylates, followed by subsequent gelatin crosslinking.41 Alternatively, a secondary material such as poly(ethylene glycol) diacrylate (PEGDA) can be used for the formation of a co-network, thereby benefitting from the higher mechanical properties of PEG, along with superior acrylatebased reaction kinetics.35
To overcome these limitations, we have developed a gelatin derivative in which all primary amines were converted to methacrylamides (0.385 mmol/g gelatin), while additional methacrylates were introduced onto the carboxylic acids. This resulted in 1 mmol of crosslinkable groups per gram gelatin (Figure 1C).5 As a consequence, a denser gelatin network was formed, exhibiting both higher stiffness and with little to no post-production swelling. Additionally, the reaction kinetics were improved compared to conventional gelatin-methacrylamide, thereby resulting in a broader 2PP spatiotemporal processing range (Figure 1A).5 Furthermore, 2D experiments indicated a comparable biocompatibility with both fibroblasts (L929) and osteoblasts (MC3T3) for Gel-MOD-AEMA and the well-established Gel-MOD.5
Although the introduction of these additional functionalities drastically improved 2PP processing, the crosslinking reactions remained subject to the drawbacks associated with chain-growth hydrogels discussed earlier. To further improve the material processing range, 2PP experiments were explored using thiolene photoclick hydrogels.22,23 In order to maximally exploit the improved reactivity of thiol-ene hydrogel systems, gelatin type B was modified to include norbornene functionalities. Gel-NB was then processed via 2PP using DTT as the thiolated crosslinker, resulting in a drastically improved spatiotemporal 2PP processing range compared to all previously reported gelatin derivatives. Only half of the energy was required to yield reproducible crosslinking (i.e. 20 mW at 100 mm/s for Gel-NB DS 63 vs 40 mW at 100 mm/s for Gel-MOD-AEMA) despite a 4 times lower concentration of crosslinkable functionalities (i.e. 0.24 mmol/g for Gel-NB vs 1 mmol/g for Gel-MOD-AEMA). Additionally, increasing the laser power above 40 mW did not influence the hydrogel swelling behaviour, which indicated that the material was already fully crosslinked. This is in contrast to Gel-MODAEMA, for which an increase of the laser power resulted in a concomitant decrease in swelling ratios.5,22 Furthermore, a broader concentration range could be applied for 2PP processing with Gel-NB, since reproducible structuring was reported for the first time below 10 w/v% gelatin concentration (i.e. 5w/v%).22 It should be noted, that Gel-NB has a significantly lower swelling ratio than Gel-MOD, due to the hydrophobic nature of the norbornene functionalities.22 As a consequence, a better computer-aided design/computer-aided manufacturing (CAD/CAM) mimicry is observed with Gel-NB, while the lower spatiotemporal energy required for full conversion leads to stiffer gels at lower laser powers. This enables the use of gel-NB for the fabrication of complex structures able to support their own weight, despite the presence of only small supporting structures (Figure 4D and E). In addition, the material can be used for the fabrication of micro-scaffolds; our studies show that scaffolds can support growth of full population by fibroblasts after 7 days of cell culture (Figure 4D).22
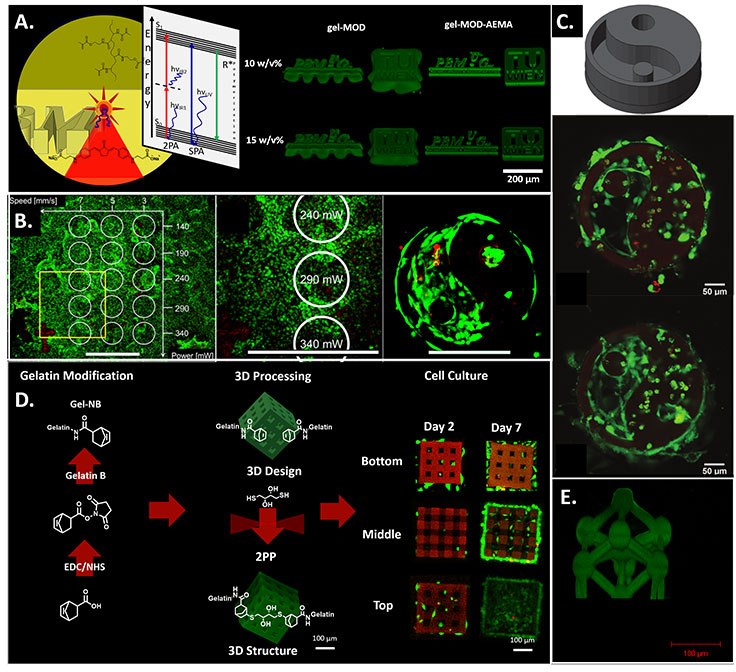
Figure 4. A) Comparison between two-photon and single-photon polymerization principle including a Jablonski diagram; Difference in shape fidelity between Gel-MOD and Gel-MOD-AEMA due to post-production swelling. Adapted with permission from reference 5, copyright 2017 ACS. B) Influence of different laser powers and scan speeds on cell viability (left and center panel, scale bars represent 1 mm). 3D structure with encapsulated MG 63 cells after 3 weeks cell culture (right panel, scale bar represents 200 μm). Adapted with permission from reference 13, copyright 2014 ACS. C) CAD image (top) of structure produced inside Gel-MOD in the presence of MC3T3 cells using a macromolecular photoinitiator. Confocal microscopy images of the structure produced inside Gel-MOD using the macromolecular two-photon photoinitiator in the presence of MC3T3 cells (center and bottom panel). Reproduced with permission from reference 42, copyright 2017 Royal Society of Chemistry. D) Scheme demonstrating the modification of gelatin into Gel-NB, 2PP structuring following a thiol-ene photoclick reaction into a microscaffold, subsequent cell culture in the presence of L929 fibroblasts after 2 and 7 days of cell culture. Reproduced with permission from reference 22, copyright 2018 Wiley. E) Atomium microstructure generated via 2PP in a 10 w/v% Gel-NB - DTT solution with an equimolar thiol/ene ratio. Image courtesy of MSc. J. Van Hoorick.
Conclusions
Throughout the past 2 decades, a plethora of photocrosslinkable gelatins suitable for tissue engineering purposes has emerged. The successful biofabrication strategies, in combination with desirable biocompatibility, cell interactivity and cost-effectiveness, have resulted in the commercialization of several photo-crosslinkable gelatin derivatives. Due to recent success with thiol-ene based systems, commercialisation of these derivatives is also anticipated soon. In addition, the combination of the availability of off-the-shelf materials, declining costs, and improved additive manufacturing technologies is likely to produce high-end biofabrication breakthroughs and subsequent integration in a clinical setting. Since gelatin is already an FDA-approved material with widespread applications in the food and pharmaceutical industry, it is only a matter of time until biofabrication strategies using photo-crosslinkable gelatins will be commonplace in the clinic.
Acknowledgement
Jasper Van Hoorick was granted an FWO-SB PhD grant provided by the Research Foundation Flanders (FWO, Belgium). The FWOFWF grant (a bilateral Research Foundation Flanders — Austrian Science Fund project) is acknowledged for financial support.
References
如要继续阅读,请登录或创建帐户。
暂无帐户?