Injectable Hydrogels for Tissue Regeneration
Prathamesh M. Kharkar1, April M. Kloxin1,2
1Materials Science and Engineering and , 2Chemical and Biomolecular Engineering, University of Delaware, Newark, DE 19716 USA
Introduction
The use of hydrogel-based biomaterials for the delivery and recruitment of cells to promote tissue regeneration in the body is of growing interest.1 Hydrogels are hydrophilic, water-swollen polymer networks formed from a variety of natural and synthetic polymeric building blocks (Figure 1). These building blocks have been engineered to enable crosslinking by chemical reaction or through physical interactions in the presence of cells and proteins that proceeds rapidly enough for injection and in situ hydrogel formation. In this article, we review principles for the design of these injectable hydrogels, advances in polymers and chemistries for their formation, and recent examples of their application for tissue regeneration.
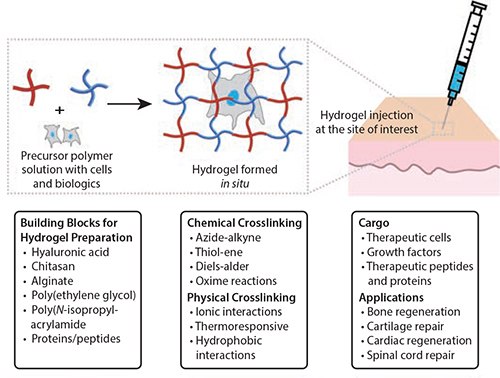
Figure 1.Injectable hydrogels for cell delivery and tissue engineering applications. Injectable hydrogels can be prepared using a variety of natural and synthetic polymers, recombinant proteins, and peptides. These base materials have been crosslinked in the presence of cells, biologics, and tissues using chemical reactions or physical interactions. Such injectable hydrogels formed in situ have been used to deliver various therapeutic cells or biologics (e.g., growth factors, chemokines for modulating the function endogenous cells) to promote the regeneration of tissues, including bone, cartilage, and skin (depicted here).
Polymeric Building Blocks for the Design of Injectable Hydrogels
Injectable hydrogels are prepared using a wide range of materials. Cyto- and bio-compatibility as well as reactive chemistries are critical factors for selecting base materials that can be used in hydrogels for cell delivery or recruitment in the body. Material crosslinking (formation and concentration of physical or covalent linkages), biodegradability, and biochemical properties are also important design criteria that can influence the structural, mechanical, and biological properties of the hydrogels initially and over time. In addition, the base polymeric materials must be stable in either solid or solution form for storage prior to use in translational or clinic studies.
The hydrophilic polymers used for hydrogel construction generally can be divided into two categories: natural polymers derived from tissues or other natural sources and synthetic polymers fabricated using organic chemistry and molecular engineering principles. Biocompatible natural polymers such as hyaluronic acid, chitosan (Prod. Nos. 448869, 448877, and 419419), heparin, alginate, fibrin (Prod. No. F5386), collagen (Prod. Nos. 804592, 804622, and 804614), chondroitin sulfate, and silk, mimic aspects of the native microenvironment, including its mechanical and biochemical properties for modulating cell adhesion, migration, and other key functions for tissue regeneration.2 These natural polymers have been used as building blocks for injectable hydrogel formation by physical (e.g., ionic, hydrogen bonding) or covalent crosslinking (e.g., reaction of functional groups on modified polymers).3 Synthetic polymers such as poly(ethylene glycol) [PEG], poly(vinyl alcohol) [PVA], poly(N-isopropylacrylamide) [PNIPAAm], and polycaprolactone [PCL] have frequently been used for the design of injectable, cell-compatible hydrogels due to their commercial availability, low batch-to-batch variation, versatility for chemical modification, and consequently, the ease of tuning the mechanical properties of the resulting hydrogels. Since synthetic polymers lack the inherent biochemical cues for interaction with cells, they are used in combination with natural polymers or biomimetic peptides to facilitate cell adhesion, migration, and protein secretion.
To capture the favorable attributes of both traditional natural and synthetic polymers, synthetic peptides and recombinant proteins have been designed for injection and subsequent assembly in situ for cell delivery. Examples of such combinations include resilin-containing proteins, tryptophan- and proline-rich sequences in mixing-induced twocomponent hydrogels (MITCH), and peptide amphiphiles with hydrophilic peptide segments (lysine or glutamic acid repeats) conjugated to a hydrophobic fatty acid.4 These designer building blocks have been engineered with integrin-binding peptide sequences to promote cell adhesion and degradable sequences for cell-driven remodeling. Here, we will focus on several polymers commonly used in designing injectable hydrogels for cell delivery and tissue regeneration.
Hyaluronic acid (HA) (Prod. No. H5542) is a non-immunogenic, biocompatible glycosaminoglycan found in the extracellular matrix of connective, epithelial, and neural tissues. HA-based biomaterials degrade in vivo in response to hyaluronidase and have been used for various biomedical applications.5 Young et al. reported use of injectable HA hydrogels for the delivery of retinal progenitor cells (RPC) for retinal repair.6 Commercially available thiol-functionalized HA was reacted with acrylate-functionalized PEG to form chemically crosslinked hydrogels in the presence of mouse retinal progenitor cells. Injected hydrogels resulted in even distribution of RPCs within subretinal space that differentiated toward desired photoreceptors. HA has been modified with a number of reactive functional groups relevant for in situ formation upon injection.7 In a recent example, Burdick et al. modified HA with aldehyde and hydrazine functional groups to create injectable hydrogels that released a recombinant tissue inhibitor of matrix metalloproteinases (TIMP3) and modulates the activities of myofibroblasts for the improved regeneration of tissue after myocardial infarction.8
Alginate, (Prod. No. 180947), a cationic biopolymer obtained from brown algae, previously has been used in several clinical applications, including as a wound dressing material. In the presence of divalent cations, alginate forms physically crosslinked hydrogels in which the mechanical properties can be tuned by varying the polymer amount, molecular weight, and the concentration of crosslinkable cations. Alginate-based injectable hydrogels have been used for the regeneration of a variety of tissues, including bone, cartilage, adipose, and cardiac tissues.9 Kim et al. demonstrated adipose tissue formation by injecting alginate hydrogels loaded with preconditioned cryopreserved human adipose stem cells (hADSCs) in vivo in mice.10 Prior to injection, alginate was oxidized to improve its biodegradability and modified using carbodiimide chemistry with an integrin-binding peptide (G4RGDASSKY) to promote cell adhesion. In 10 weeks, hydrogels containing hADSCs resulted in the formation of adipose tissue (~50% of initial gel volume), where a negative control (hydrogels without cells) lacked new tissue formation. In addition to HA and alginate, injectable hydrogels have been prepared using a variety of other natural polymers, including chitosan, heparin, collagen, gelatin, chondroitin sulfate, or combinations of these, for cartilage, bone, and cardiac tissue regeneration.11
PEG is one of the most widely used synthetic polymers for the preparation of injectable hydrogels due to its biocompatibility and lack of protein binding sites. PEG provides a relatively bioinert base for the introduction of specific bioactive groups (e.g., peptides) to modulate interactions with cells and has been modified with a variety of end groups, including amines, thiols, maleimides, acrylates, and norbornenes, that are easily synthesized or obtained commercially.12 Several research groups including ours have developed PEG-based hydrogels for cell delivery and tissue regeneration. For example, Garcia et al. recently used PEG macromers functionalized with maleimides and peptides functionalized with thiols (i.e., cysteines) to form injectable hydrogels for cell delivery.13 These hydrogels maintained the viability of progenitor cells (C2C12s) (Prod. No. 91031101) during encapsulation, where crosslinking times ranged between 1–5 minutes based on the polymer concentration, and promoted cell spreading.
Crosslinking and Degradation Reactions for Controlling Hydrogel Formation and Erosion
Injectable hydrogels are formed using a variety of chemical or physical crosslinking strategies which must be carefully selected to match the specific application of interest. For example, an appropriate crosslinking rate is essential for the proper formation of an injectable hydrogel in situ. If the gelation is too slow, precursors are likely to perfuse from the site of injection into surrounding tissues, leading to poor hydrogel properties and potentially to an inflammatory response to unreacted monomers. If the gelation is too rapid, shear thinning (and potentially premature gel formation) in the syringe may occur, introducing network defects that, in turn, affect gel mechanical properties and retention/release of cargo (i.e., cells). In addition, the ability to form the hydrogel in the presence of live cells and therapeutic proteins is the key for cell delivery and regenerative medicine applications. The following discussion will highlight a few relevant crosslinking techniques with recent examples.
Due to its rapid crosslinking kinetics and high efficiency, click chemistry represents one of the most attractive classes of crosslinking chemistries for the formation of hydrogels for cell delivery. Commonly used copper catalyzed alkyne-azide click reactions have been utilized to form hydrogels;14 however, copper can be cytotoxic and its use limits applicability in cell encapsulation and delivery applications. To overcome this, strain promoted azide-alkyne cycloadditions (SPAAC) have been utilized to crosslink cytocompatible hydrogels. DeForest and Anseth reported the use of SPAAC for fibroblast encapsulation in hydrogels.15 The authors showed that when a four-arm PEG end-functionalized with difluorinated cyclooctyne is reacted with an azide-functionalized peptide crosslinker, the ring strain and electron-withdrawing fluorine substituents promote a rapid crosslinking reaction (~2 minutes) without a catalyst. In another approach, Dove et al. recently reported the catalyst-free reaction of azide-functionalized chitosan with propionic acid-functionalized PEG to form hydrogels in the presence of cells.16 The authors were able to encapsulate mesenchymal stem cells during hydrogel formation and showed the encapsulated cells exhibit high viability after 24 hours of culture (>95%). These and other click reactions, including thiol–enes (in particular, Michael additions of thiols with maleimides or vinyl sulfones), Diels-Alder, aldehyde-hydrazine, and oxime reactions, are attractive for injectable hydrogel applications. For a comprehensive summary of chemical crosslinking reactions, readers are referred to recent review articles.3,12,17
Physically crosslinked injectable hydrogels have been formed using ionic, hydrophobic, hydrogen bonding, or guest-host interactions, most commonly through thermoresponsive and ionic interactions.18 Reis et al. reported formation of injectable hydrogels using thermoresponsive poly(N-isopropylacrylamide)-g-methylcellulose (PNIPAAm-g-MC) for cartilage tissue engineering applications.19 Hydrogel formation was achieved using the thermoresponsive phase transition of the PNIPAAmg- MC polymer with gelation occurring above its lower critical solution temperature (~32 °C). Encapsulated ATDC5 chondrogenic cells adopted a rounded morphology, supporting maintenance of the chondrocyte phenotype, which is critical for articular cartilage regeneration. Physical crosslinking using ionic interactions is extensively used to crosslink polysaccharides such as alginate and chitosan. For example, Xu et al. encapsulated human umbilical cord mesenchymal stem cells (hUCMSCs) in alginate-based injectable hydrogels for bone tissue engineering.20 Gelation of alginate was achieved by crosslinking with calcium, a divalent cation in the presence of hUCMSCs with a viability of ~70% post-encapsulation.
For all cell delivery and tissue regeneration applications, hydrogels must degrade in a controlled manner after injection for the desired therapeutic use (e.g., cell release or infiltration, protein elaboration) without cytotoxic degradation byproducts. Typically, degradation can be achieved using cleavable polymeric backbones or dynamic/reversible crosslinks. As mentioned earlier, natural polymers such as hyaluronic acid and alginate undergo enzymatic hydrolysis in vivo, providing a mechanism for hydrogel degradation. For synthetic polymers, hydrogels have been traditionally engineered with ester linkages like poly(lactic acid) for degradation by ester hydrolysis in aqueous microenvironments preprogrammed based on the number of esters incorporated. In addition, several research groups have explored additional responsive or triggerable degradable chemistries, including photodegradation,21 retro Michael type,22 and retro Diels-Alder reactions,23 for precise control over material degradation in situ. More recently, in collaboration with the Kiick research group, we have achieved precise control over the degradation of injectable hydrogels formed with thiol-maldeimide chemistry by incorporating two or more modes of degradation using combinations of these chemistries.24 Hydrogel degradation over multiple time scales was achieved by incorporation of: i) o-nitrobenzyl ether linkages that undergo photodegradation (k ~ 10–1 min–1 for 365 nm to 10–2 min–1 for 400–500 nm); ii) aryl-thiol-based succinimide thioether linkages that undergo retro Michael-type reaction in the presence of glutathione (k ~ 10–3 min–1); and iii) ester linkages that undergo ester hydrolysis in aqueous microenvironments (k ~ 10–4 min–1). In principle, such injectable hydrogels with multimodal degradability could be utilized to deliver or recruit cells with precise and tailorable control over gel degradation and release profiles for tissue regeneration.
Cell Delivery and Tissue Regeneration with in situ Forming Hydrogels
Injectable hydrogels have been used to deliver a variety of cargo molecules, including growth factors, chemokines, and therapeutic cells to promote tissue regeneration in vitro and in vivo. These in situ forming materials are being used for the regeneration of a variety of tissues, including bone, cartilage, intervertebral disc, adipose, cardiac, and vascular tissues. A few recent examples of injectable hydrogels used for bone, cartilage, and cardiac tissue regeneration are highlighted here. A comprehensive review of this growing research area can be found in recent a review article.25
Bone
Addressing bone defects and abnormalities is one of the key challenges in orthopedics. Bone tissue engineering strategies using injectable hydrogels provide an attractive alternative to traditional treatments, such as autologous bone implantation and bone graft materials, as demonstrated in seminal works by Hubbell et al.26 Recently, Song et al. investigated the use of injectable thermoresponsive poly(phosphazene)- based hydrogels for sustained delivery of bone morphogenetic protein-2 (BMP-2) (Prod. No. H4791).27 Poly(phosphazene) with additional carboxylic groups, which promote ionic interactions with BMP-2, were used to form hydrogels upon injection, where gelation occurs as the temperature approaches physiological temperature (37 °C). Prolonged release of BMP-2 from such hydrogels over 20 days was achieved in vitro and resulted in significantly higher osteocalcin secretion (~14 ng/mL) by C2C12 cells (Prod. No. 91031101) compared to the control (~2 ng/mL), suggesting retention of the bioactivity of BMP-2 for osteoblastic differentiation. Injection of the BMP-2 loaded hydrogels in vivo resulted in ectopic bone formation in mice, highlighting the promise of injectable hydrogels for bone regeneration.
Cartilage
Due to the limited self-healing capacity of cartilage tissues, a variety of surgical treatments have been developed to promote cartilage regeneration. Among these, use of injectable or in situ forming hydrogels to deliver growth factors and therapeutic cells at the site of the cartilage defect is one emerging strategy.28 Jin et al. reported injectable and biodegradable hydrogels formed using dextran and hyaluronic acid for cartilage tissue engineering. Rapid hydrogel formation (~2 minutes) was achieved using oxidative coupling of phenolic groups in the presence of horseradish peroxidase (Prod. No. 77332) and hydrogen peroxide (H2O2, Prod. No. H3410). Bovine chondrocytes maintain high cell viability during the encapsulation process (~95%) and exhibit increased glycosaminoglycan and collagen production over 21 days. For these types of repairs, surgery is requisite for accessing the tissue site. Consequently, other reactive chemistries that enable rapid, triggered gel formation in situ also have been utilized (e.g., photopolymerized methacrylate or thiol–ene systems29) and represent active areas of research for tissue regeneration in non-uniform defect sites. Such in situ forming hydrogels are promising for the repair of cartilage defects.
Cardiovascular Tissues
Cardiovascular disease is another challenging area where injectable hydrogels offer promising treatment options. To address challenges associated with chronic myocardial infarction, Park et al. developed thiol– ene hydrogels that use acrylated hyaluronic acid and a thiol-containing MMP-sensitive peptide crosslinker to modulate cellular activities toward tissue regeneration.30 Specifically, a chemokine, stromal-derived factor-1, (SDF-1) (Prod. Nos. SRP3253 and SRP3276) that plays a key role in repair of myocardial infarction and Ac-SDKP (a peptide that stimulates endothelial cell proliferation and angiogenesis) were incorporated within the hydrogel precursor solution. Protein-laden hydrogels were formed upon injection into the epicardium in a myocardial infarction model and heart functions were evaluated after 4 weeks. These evaluations showed that injection of SDF-1 and SDKP laden hydrogels improved ventricle function, increased angiogenesis, decreased infarct size (~8% for SDF-1/SDKP compared to ~41% for control), and increased wall thickness (211% higher compared to control) within the infarct region. These results demonstrate the utility of injectable hydrogels for modulating cellular functions, including endogenous cells, for tissue regeneration.
Conclusion
Over the past decade, injectable hydrogels have emerged as promising biomaterials for cell delivery or recruitment for promoting tissue regeneration. Significant advancements have been made in the design of the base polymers and crosslinking chemistries for hydrogel formation and the cleavable and reversible chemistries for hydrogel degradation, both in the presence of cells and tissues. These approaches maintain the bioactivity of delivered therapeutic cells and biologics and are leading to advances in the quality and function of regenerated tissues.
Acknowledgments
The authors gratefully acknowledge support for related work from the Delaware COBRE program with a grant from the National Institute of General Medical Sciences (NIGMS P20GM104316-02), from the National Institutes of Health, the Burroughs Wellcome Fund, and the University of Delaware Research Foundation. We thank the MediaLab in the Department of Biochemistry at the University of Wisconsin at Madison for the use of an Adobe Illustrator template.
Product No. | Product Name | Description | Pricing |
---|---|---|---|
697230 | [1,1′-双(二苯基膦)二茂铁]二氯化钯(II) | ||
151076 | 9-硼双环[3.3.1]壬烷 溶液 | 0.5 M in THF | |
296090 | 正己烷 | anhydrous, 95% | |
401757 | 四氢呋喃 | anhydrous, ≥99.9%, inhibitor-free |
References
To continue reading please sign in or create an account.
Don't Have An Account?