The Carbon Enigma: Material Fundamentals and Retention Properties for Porous Graphitic Carbon (PGC) Stationary Phases
Clinton Corman, HPLC R&D Sr. Scientist, William Maule, HPLC R&D Sr. Scientist, Michael Ye, HPLC R&D Manager, Cory Muraco, Global Product Manager Liquid Chromatography Technologies
Merck

Introduction
Despite its uniqueness compared to more conventional liquid chromatography (LC) stationary phases, Porous Graphitic Carbon (PGC) has been around since the 1980’s. During that time, the goal was to generate a material that had the advantages of standard Reversed- Phase (RP) phases based on silica supports while eliminating some of the negative attributes, such as limited pH range, limited temperature stability, and secondary interactions from active surface silanols. What the researchers discovered was while there is some overlap with standard RP phases, some degree of graphitization gives the material other distinctive properties. Due to some of these characteristics specific to graphites, PGC has situated itself as more of a specialty phase for more challenging separations when other more traditional options do not work.
Brief History Lesson
The first published papers on the topic of PGC date back to 1982 when Gilbert et al demonstrated the phase’s usefulness in both Gas Chromatography (GC) and Liquid Chromatography (LC).1 At the time, this material was referred to as Porous Glassy Carbon.
Although the terminology is somewhat different, it is indeed very similar, and somewhat appropriate terminology, to describe the commercial PGC materials used in HPLC columns today. From here, the processing of this material was studied and optimized further by Professor John Knox and others at the University of Edinburgh,2 leading to a material that was suitable for packing in HPLC columns. Since then, another PGC stationary phase material is available, Supel™ Carbon LC, which, albeit, has many similarities to pre-existing PGC phases, but has some differences as well.
Particle Morphology
In order to aid in understanding how retention is governed on a PGC stationary phase for liquid chromatography, some general prerequisite information about the material is needed. Graphite is one of the many allotropes of carbon. Graphite consists of stacked planes of six-membered carbon rings with each carbon atom arranged in a hexagonal or honeycomb-like lattice within each individual plane. The individual planes are referred to as graphene while graphite is essentially stacked layers of graphene planes (Figure 1).
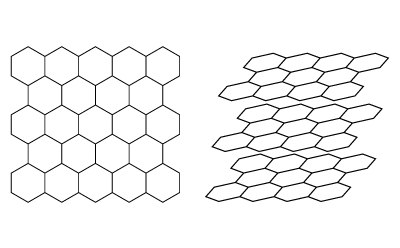
Figure 1.(Left) Hexagonal arrangement on a single plane of graphite. (Right) Stacked layers of graphene forming graphite.
Within a plane, each carbon atom is covalently bonded to three adjacent carbon atoms, but since carbon has four valence electrons available for bonding, the remaining valence electron is transposed perpendicular above or below the plane in p-orbitals (Figure 2).

Figure 2.p-Orbitals on each carbon atom results in sp2 hybridization.
Since every carbon atom in the plane has a delocalized electron in its p-orbital, this overlap results in sp2 orbital hybridization where all the delocalized electrons are free to move in the plane resulting in a continuous electron cloud called a pi-orbital. This phenomenon is sometimes referred to as the pi-cloud of graphite.
The layers are held together by weak van-der-Waals forces and, depending on the layer registration, the graphite can be considered two-dimensional or three-dimensional. Three-dimensional graphite has an ordered layer registration such as ABAB or ABCABC while two-dimensional graphite does not have specific ordering and the planes do not have perfect spacing and alignment with each other within the stacks.2 This poor arrangement between layers is often described as turbostratic. While three-dimensional graphite exists, the PGC particles developed for chromatographic purposes have all been two-dimensional graphites as consequence of how the material is produced. The original term “Glassy” carbon which was later changed to “Graphitic” Carbon is worth noting because typically glassy carbons are produced from the pyrolysis of phenolic resins or similar resins under extreme heating, sometimes above 3000 °C which is not too dissimilar to current commercialized PGC phases. However, glassy carbons are non-graphitizable materials and no amount of heating will cause any degree of crystalline graphite formation. PGC, on the other hand, has some degree of crystalline graphite. PGC is generated from an amorphous carbon template on extreme heating at temperatures above 2000 °C. This causes a carbon atom rearrangement to occur, forming graphitic layers in the form of ribbons that randomly become intertwined.2 This weaving of graphitic ribbons is a primary distinction between a typical graphitic carbon black (GCB) and PGC and lends itself to why PGC has the durability to be used in HPLC whereas GGCBs do not. When magnified for perspective, as can be seen in the example illustrated in Figure 3 a-c, the top surface of PGC is not completely flat but consists of sections of exposed graphitic ribbons that eventually end and meet adjacent graphitic planes that are part of the same or a different intertwined graphitic ribbon. To put it simply, the surface can be visualized as having flat sections and edges where flat sections converge against one another.
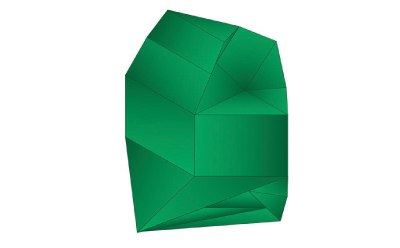
Figure 3a.Zoomed in illustration of the different graphitic planes on the top surface of PGC.
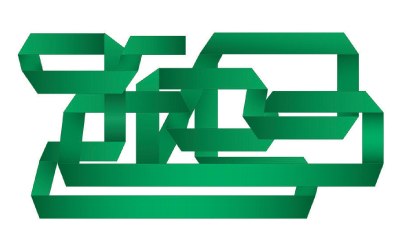
Figure 3b.The intertwined graphitic ribbons that give PGC particles its robust strength.
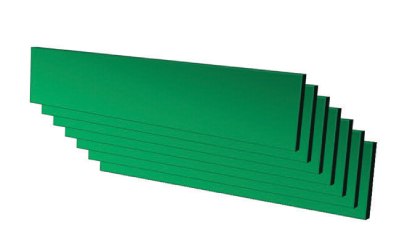
Figure 3c.A cut section of a graphitic ribbon exposing multiple layers.
It has been hypothesized that the edge planes may play a role in PGC’s chromatographic behavior since there is potential, unfilled valency on the edge carbons. Previous work downplays the importance of functional groups on the edge planes.1,2,3 However, one study theorized that edge planes may have a more critical role than initially thought and played a part in the oxidation of the stationary phase which resulted in altered retention characteristics.4 Another theory is that the electron distribution on the graphitic plane favors localization near the extremities or edges resulting in a partial positive or neutral center and a progressively increasing negative charge towards the outermost edges resulting in analytes interacting in specific regions on the graphitic surface based on properties such as formal charge.5,6 There are still unanswered questions in this respect, but further modeling and characterization are needed to enhance the current prevailing theories.
Retention Properties
Many theoretical studies have been done to better understand the interactions that take place on a PGC surface and drives analyte retention in liquid chromatography. Unfortunately, most of the studies use different mobile phase setups with a wide variety of buffers and additives, thus further complicating the situation. This attribute becomes especially challenging because some additives that are commonly used with PGC adsorb strongly to the PGC surface, altering its chromatographic behavior. Nevertheless, these fundamental studies have helped reach some logical conclusions. To briefly summarize, as it is understood thus far, retention is governed by multiple competing factors:
- Dispersive Interactions – Similar in behavior to that seen in reversed phase chromatography and heavily driven by mobile phase strength (organic/aqueous ratio) and analyte properties.7-10
- Solvent/Solute interactions that form in a solution and can either be dipole-dipole or H-bonding or hydrophobic-hydrophilic repulsion interactions.
- Solvent & solute dispersive forces (London) with graphite.
- Electronic Interactions between polarizable groups of the analyte and the surface of graphite (often referred to as PREG – polar retention effect on graphite).2,7,8,11
Not as well understood but is thought to stem from some form of charge induction or electron lone pair donating/accepting interactions with the pi cloud of graphite. This effect can be significant if there is pi-pi overlap due to unsaturation or aromaticity of the analyte. Likewise, the stereochemistry of the analyte plays an important role and the location of the polar functional groups with respect to the graphitic plane. - Retention is heavily influenced by the analyte size and shape whereby increased surface area contact of the analyte with the surface of graphite results in longer retention times than minimal surface area contact. This trait becomes especially noticeable with aromatic compounds (pi-pi overlap seems to be especially important with aromatics).
Interaction with Hydrophobic Analytes
In theory, a surface comprising of all carbon atoms should be very hydrophobic and will behave like a long alkyl-chain, reversed-phase support, but this is not always the case for PGC. We examined a set of substituted alkyl benzenes and nitroalkanes on both a C18 column and a PGC column (Figure 4 & Table 1). In general, the compounds behave similarly but there are some differences as well.
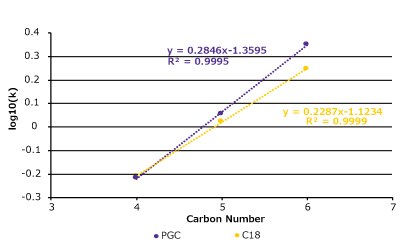
Nitroalkanes

Alkylbenzenes
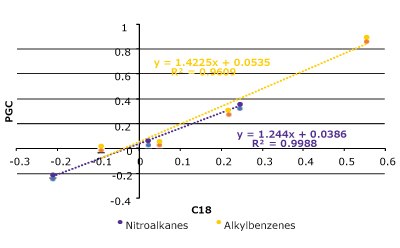
Figure 4. Hydrophobicity comparison between PGC and C18 with a set of nitroalkanes and alkylbenzenes. (Top) A series of nitroalkanes & alkyl benzenes log(k) data plotted against its substituent's carbon number on a PGC and C18 column. (Bottom Left) k-k Plot of log(k) data on both PGC & C18 which displays strong correlation (R2 = 0.96). (Bottom Right) Analyte structures (see Table 1 for conditions).
Column: | Ascentis® Express C18 50 x 3 mm I.D., 2.7 μm (53811-U); Supel™ Carbon LC 50 x 3 mm I.D., 2.7 µm (59991-U) |
Mobile phase: | [A] Acetonitrile; [B] Water; (80/20 A/B for alkylbenzenes, 60/40 A/B for nitroalkanes) |
Flow rate: | 0.5 mL/min |
Injection Volume: | 1.0 µL |
Column temperature: | 25 °C |
Detector: | UV, 190 nm & 210 nm |
Sample: | Alkylbenzenes – toluene, ethylbenzene, propylbenzene, pentylbenzene, 100 μg/mL ea. in mobile phase; Nitroalkanes – 1-nitrobutane, 1-nitropentane, 1-nitrohexane 100 μg/mL ea. in mobile phase; Urea (t0 marker) 1000 μg/mL in mobile phase |
The C18 column and the PGC column perform similarly, and the k-k plot confirms that the relationship between these two phases across the two compound sets is quite linear. The homologous series plot shows that the PGC column has a slightly steeper slope indicating that it is more selective towards each methylene addition compared to the C18 column. Interestingly, for the alkylbenzenes the methylene addition from C1 (toluene) to C2 (ethyl benzene) does not follow the same slope pattern as the rest of data set. The reasoning for this outcome may be related to toluene's aromatic shape and the fact that ethyl benzene has free rotation and loses some molecular surface area that can contact the stationary phase surface.
Interaction with Hydrophilic Analytes
PGC is known for its ability to retain more polar, hydrophilic analytes that typically are unretained on a standard, reversed-phase column such as C18. However, there is some confusion as to which type of compounds will and will not retain. We examined two sets of polar analytes.
As can be seen from the chromatographic results in Figure 5 (conditions in Table 2), the C18 column is only able to retain acetone. However, on the PGC column, all the analytes in set two are retained and only acetone is slightly retained from set one. For the C18 column, it makes sense that it cannot retain these analytes. The compounds are very hydrophilic with negative log P values hydrophilic and the interaction of the octadecyl ligand is not strong enough to break the even stronger dipole-dipole interactions that these polar analytes are involved in with the bulk mobile phase (90% water / 10% acetonitrile). Thus, the analytes stay in solution and no retention is observed.

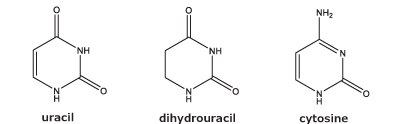
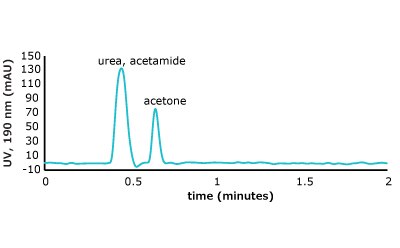


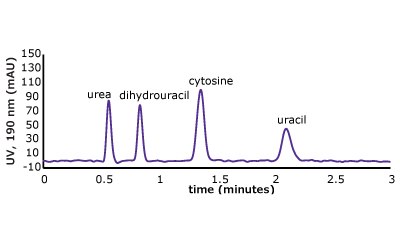
Figure 5. (Top) Set 1 & 2 analyte structures, (Middle) Set 1 & 2 chromatograms on C18, (Bottom) Set 1 & 2 chromatograms on PGC. In this case, the C18 column cannot retain any of the analytes except acetone. Conversely, the PGC column is able to retain cytosine and uracil from set 2, but no or poor retention of set 1 analytes is observed similar to the C18 performance (see Table 2 for conditions).
Column: | Ascentis® Express C18 50 x 3 mm I.D., 2.7 μm (53811-U); Supel™ Carbon LC 50 x 3 mm I.D., 2.7 µm (59991-U) |
Mobile phase: | [A] Acetonitrile; [B] Water; (10/90 A/B) |
Flow rate: | 0.5 mL/min |
Injection Volume: | 1.0 µL |
Column temperature: | 25 °C |
Detector: | UV, 190 nm & 210 nm |
Sample: | Set 1 – Urea, Acetamide, Acetone, 100 μg/mL ea. in mobile phase; Set 2 – Urea, Dihydrouracil, Cytosine, Uracil, 100 μg/mL ea. in mobile phase |
The analyte interactions with the PGC column are completely different. For set one, it seems that for small, polar analytes, poor retention is observed similar to the C18 phase. All three analytes are planar, have pi electrons due to the carbonyl group, and have an uneven charge distribution. These are good analyte criteria for retention on PGC. While this is all true, it seems that, for very small polar molecules, the uneven charge distribution is either not inducing the graphitic surface, or its contribution is not noticed because it is superseded by the mobile phases’ proclivity to keep the solutes in solution. Additionally, with small, polar molecules, there is not enough molecular surface area to interact with the graphitic plane when compared to a much larger polar molecule. Contrast that with the set two compounds, the polar, cyclic compounds, which can be retained. Uracil is a common t0 marker in reversed-phase chromatography, but this cannot be used as such on PGC-based materials. Cytosine, which is even more hydrophilic than uracil is also retained. The most compelling detail about the elution order is the significantly earlier elution of dihydrouracil than uracil.
The Importance of Analyte Stereochemistry
It has been well documented over the years that PGC exhibits unique shape selectivity for compounds based on their stereochemistry. Even some of the prior qualitative examples shown glean of some aspects of this phenomenon. Why did the slope for the alkylbenzenes series improve in linearity when toluene was removed from the data set? Likewise, why does dihydrouracil show significantly less retention? Examining the latter option, uracil has a completely planar structure but losing the double bond in the ring the molecule has to have some free rotation and dihydrouracil to be slightly bent. As a result, dihydrouracil is non-planar and cannot position as much surface area onto the graphitic plane compared to uracil (Figure 6). This aspect is an important factor that must be considered when using PGC as a stationary phase as analyte stereochemistry is a driving factor as to why PGC can retain, cannot retain, or discriminate similar compounds.

Figure 6.An illustration of how analyte shape affects how much maximal molecular surface area contact happens on the graphitic surface of PGC. Notice the larger shadow for the compound on the left versus the compound on the right, indicating more surface area interaction and stronger interaction with the stationary phase.
Conclusions
Porous graphitic carbon (PGC) is a unique stationary phase giving the chromatographer an additional chemistry option to separate challenging compounds beyond the realm of conventional reversed-phase chromatography. In many respects, the PGC column may behave like a reversed-phase column with enhanced temperature, and pH stability, but due to the special properties of graphite, polar compounds that may need HILIC or ion-exchange conditions can be retained as well. However, some aspects of the analyte’s stereochemistry and potential surface area contact points need to be considered for there to be enough retention. Although the mechanisms are not fully understood, especially in regards to PREG, it is undeniable that PGC has unique retention properties towards polar compounds – especially planar molecules or analytes with double bond conjugation that can interact with the electron cloud of graphite. More research into these fundamental mechanisms will yield more accurate retention prediction models.
References
To continue reading please sign in or create an account.
Don't Have An Account?